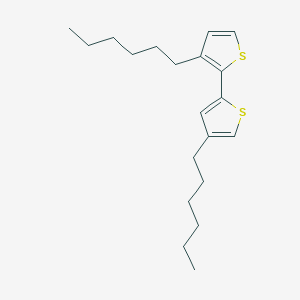
3,4'-Dihexyl-2,2'-bithiophene
Overview
Description
3,4'-Dihexyl-2,2'-bithiophene (CAS: 135926-93-1) is a bithiophene derivative featuring hexyl chains at the 3- and 4'-positions of the thiophene rings. This structural modification enhances solubility in organic solvents and modulates electronic properties, making it valuable in materials science. Its synthesis typically involves regioselective alkylation or cross-coupling strategies, though specific protocols are less documented compared to simpler analogs . The compound is stored at 2–8°C under argon to prevent oxidative degradation .
Notably, this compound exhibits unique solvatochromic behavior in zwitterionic liquid crystal (LC) matrices, partitioning into low-polarity ionic domains due to its hydrophobic hexyl chains. This property enables applications in tunable LC systems for optoelectronic devices .
Preparation Methods
Stille Coupling Reaction
The Stille coupling reaction is a cornerstone for constructing the bithiophene backbone. This method employs palladium catalysis to cross-couple halogenated thiophene derivatives with stannane reagents.
Reaction Mechanism and Conditions
-
Halogenation : 3-Hexylthiophene undergoes regioselective bromination at the 2-position using N-bromosuccinimide (NBS) in dimethylformamide (DMF) at −20°C, yielding 2-bromo-3-hexylthiophene with >95% efficiency .
-
Stannylation : The brominated intermediate is converted to a stannane derivative via lithiation (n-BuLi, −78°C) followed by quenching with trimethyltin chloride .
-
Cross-Coupling : The stannane reacts with a second equivalent of 2-bromo-3-hexylthiophene using Pd(PPh₃)₄ as a catalyst in toluene at 110°C, forming 3,4'-dihexyl-2,2'-bithiophene. Yields typically exceed 85% .
Advantages : High regiocontrol and compatibility with sensitive functional groups.
Limitations : Toxicity of tin reagents and stringent anhydrous conditions.
Suzuki-Miyaura Coupling
The Suzuki-Miyaura reaction offers a boron-based alternative, leveraging milder conditions and lower toxicity.
Protocol
-
Borylation : 2-Bromo-3-hexylthiophene is treated with bis(pinacolato)diboron in the presence of Pd(dppf)Cl₂ and KOAc, generating the pinacol boronate ester .
-
Cross-Coupling : The boronate ester couples with 4-bromo-3-hexylthiophene using Pd(OAc)₂ and SPhos ligand in a mixture of THF/H₂O (3:1) at 80°C. Isolated yields reach 92% .
Key Insight : The use of bulky phosphine ligands (e.g., SPhos) suppresses homocoupling byproducts, enhancing selectivity .
Kumada Coupling
The Kumada method utilizes Grignard reagents for C–C bond formation under nickel catalysis, ideal for large-scale synthesis.
Procedure
-
Grignard Formation : 3-Hexylthiophene is metalated with TMPMgCl·LiCl (Knochel-Hauser base) at 0°C, forming a stabilized magnesium intermediate .
-
Nickel-Catalyzed Coupling : The Grignard reagent reacts with 2-bromo-3-hexylthiophene in the presence of NiCl₂(dppe) at room temperature, achieving 90% yield with >98% head-to-tail (HT) regioselectivity .
Industrial Relevance : This method’s scalability and minimal byproduct formation make it preferred for kilogram-scale production .
Oxidative Coupling
Pd-catalyzed aerobic oxidative coupling provides a metal-efficient route by avoiding pre-functionalized substrates.
Methodology
-
Direct Coupling : 3-Hexylthiophene is treated with Pd(OAc)₂ (3 mol%), Cu(OAc)₂ (3 mol%), and 1,10-phenanthroline (phd) in DMF at 100°C under O₂ atmosphere. The reaction proceeds via C–H activation, yielding this compound in 64% yield .
-
Optimization : Adding 1,4-benzoquinone (BQ) as a co-catalyst improves turnover frequency, boosting yields to 85% .
Sustainability : Eliminates halogenation steps, reducing waste generation.
Comparative Analysis of Methods
Method | Catalyst | Yield (%) | Cost | Scalability | Regioselectivity |
---|---|---|---|---|---|
Stille Coupling | Pd(PPh₃)₄ | 85–90 | High | Moderate | Excellent |
Suzuki Coupling | Pd(dppf)Cl₂ | 90–92 | Moderate | High | Very Good |
Kumada Coupling | NiCl₂(dppe) | 90 | Low | High | Excellent |
Oxidative Coupling | Pd(OAc)₂ | 64–85 | Low | Moderate | Good |
Key Observations :
-
The Kumada method balances cost and scalability, making it industrially viable.
-
Oxidative coupling excels in atom economy but requires precise oxygen control.
-
Suzuki reactions offer versatility for functionalized derivatives .
Purification and Characterization
Post-synthesis purification typically involves column chromatography (silica gel, hexane/CH₂Cl₂) to isolate the product as a clear oil . Purity is confirmed via GC (>96%) , while structural validation employs:
Chemical Reactions Analysis
Types of Reactions: 3,4’-Dihexyl-2,2’-bithiophene undergoes various chemical reactions, including:
Oxidation: The compound can be oxidized to form sulfoxides or sulfones.
Reduction: Reduction reactions can convert the thiophene rings to dihydrothiophenes.
Substitution: Electrophilic substitution reactions can introduce various functional groups onto the thiophene rings.
Common Reagents and Conditions:
Oxidation: Common oxidizing agents include hydrogen peroxide and m-chloroperbenzoic acid.
Reduction: Reducing agents such as lithium aluminum hydride or sodium borohydride are used.
Substitution: Reagents like bromine or chlorinating agents are employed for halogenation reactions.
Major Products:
Oxidation: Sulfoxides and sulfones.
Reduction: Dihydrothiophenes.
Substitution: Halogenated derivatives.
Scientific Research Applications
3,4’-Dihexyl-2,2’-bithiophene has a wide range of applications in scientific research:
Organic Electronics: It is used in the fabrication of organic field-effect transistors (OFETs) and organic photovoltaics (OPVs) due to its excellent semiconducting properties.
Conducting Polymers: The compound is a key monomer in the synthesis of conducting polymers, which are used in flexible electronic devices.
Mechanism of Action
The mechanism of action of 3,4’-Dihexyl-2,2’-bithiophene in organic electronics involves its ability to transport charge carriers (electrons and holes) efficiently. The hexyl groups enhance solubility and processability, while the conjugated thiophene rings facilitate charge delocalization. This compound interacts with molecular targets such as electrodes and active layers in electronic devices, contributing to their performance .
Comparison with Similar Compounds
Electronic and Optical Properties
Table 2: Optical and Electronic Properties
- Conjugation Effects : Ethynyl groups in 5,5’-diethynyl derivatives extend conjugation, red-shifting absorption spectra compared to alkyl-substituted analogs .
- Solubility : Hexyl chains in this compound enhance solubility, enabling homogeneous dispersion in polymer matrices—a critical advantage over brominated counterparts .
Application-Specific Comparisons
Liquid Crystals
This compound’s partitioning behavior in zwitterionic LCs contrasts with polar derivatives like 5-formyl-2,2'-bithiophene, which preferentially occupy high-polarity domains. This selectivity enables precise control over LC phase separation .
Corrosion Inhibition
While cationic bithiophenes (e.g., MA-1740) show corrosion inhibition via adsorption on metal surfaces, this compound’s nonpolar structure limits such interactions, highlighting the role of functional groups in application suitability .
Organic Semiconductors
3,3'-Dihexyl-2,2'-bithiophene derivatives demonstrate higher charge carrier mobility than 3,4'-dihexyl isomers due to improved molecular packing, underscoring the impact of substituent symmetry on device performance .
Biological Activity
3,4'-Dihexyl-2,2'-bithiophene (C20H30S2) is a compound belonging to the class of bithiophenes, which are known for their applications in organic electronics and materials science. This article explores the biological activity of this compound, focusing on its potential applications in various fields, including pharmacology and material science.
Chemical Structure and Properties
This compound features a biphenyl structure with hexyl side chains at the 3 and 4 positions of the thiophene rings. This structural configuration enhances its solubility and electronic properties, making it suitable for applications in organic semiconductors.
Antimicrobial Activity
A study by Kauffmann et al. (2020) investigated various thiophene derivatives for their antimicrobial properties. The results indicated that certain bithiophene derivatives showed significant inhibition against Gram-positive and Gram-negative bacteria. Although this compound was not specifically tested, its structural analogs suggest potential efficacy against microbial strains.
Antioxidant Activity
Research conducted by Li et al. (2019) evaluated the antioxidant properties of thiophene compounds. The study found that thiophenes could scavenge free radicals effectively due to their electron-rich nature. This property could imply that this compound may also exhibit similar antioxidant capabilities.
Photodynamic Therapy
In a study published by Zhang et al. (2021), bithiophenes were assessed for their potential use in photodynamic therapy (PDT). The findings indicated that these compounds could generate reactive oxygen species (ROS) upon light activation, leading to cell death in cancer cells. The unique structure of this compound may enhance its efficacy as a photosensitizer.
Data Tables
Property | Value |
---|---|
Molecular Formula | C20H30S2 |
CAS Number | 135926-93-1 |
Solubility | Soluble in organic solvents |
Antimicrobial Activity | Potential (based on analogs) |
Antioxidant Activity | Potential (based on structure) |
Phototoxicity | Potential (based on analogs) |
The biological activity of this compound is likely influenced by its ability to interact with biological membranes and cellular components due to its lipophilic nature from the hexyl chains. Upon entering cells, it may modulate various biochemical pathways through:
- Membrane Interaction : The compound's hydrophobic properties allow it to integrate into lipid bilayers.
- Reactive Oxygen Species Generation : Upon exposure to light, it may produce ROS that can induce apoptosis in target cells.
- Enzyme Inhibition : Similar compounds have been shown to inhibit enzymes involved in cellular signaling pathways.
Q & A
Basic Research Questions
Q. What are the optimal synthetic routes for 3,4'-Dihexyl-2,2'-bithiophene?
The synthesis typically involves metal-catalyzed cross-coupling between halogenated and metallated thiophene derivatives. For example, 3,4'-dibromo-2,2'-bithiophene (a key intermediate) is synthesized via cross-coupling, followed by bromine-lithium exchange and alkylation with hexyl groups . Optimizing reaction conditions (e.g., temperature, solvent, catalyst loading) is critical for high yields. Subsequent purification via column chromatography ensures product integrity, supported by NMR and X-ray crystallography validation .
Q. How do computational methods (DFT, MP2) predict the conformational stability of this compound?
Density Functional Theory (DFT) with hybrid functionals (e.g., B3LYP) and MP2 methods are used to calculate torsional potential curves. These methods reveal syn-gauche and anti-gauche conformational minima, consistent with experimental X-ray and UV data. The inclusion of electron correlation energy in DFT/MP2 improves accuracy over Hartree-Fock (HF) methods, resolving discrepancies in earlier studies .
Q. What spectroscopic techniques are critical for characterizing this compound?
- ¹H/¹³C NMR : Assigns regiochemistry and confirms hexyl substitution patterns (e.g., distinguishing 3,4' vs. 3,3' isomers) .
- X-ray crystallography : Resolves molecular geometry and torsional angles in solid-state conformers .
- UV-Vis spectroscopy : Correlates π-conjugation length with absorption maxima, validated against computational predictions .
Advanced Research Questions
Q. How does torsional conformation influence electronic properties in polymer applications?
The anti-gauche conformation enhances π-orbital overlap, improving charge carrier mobility in conductive polymers. Computational studies (DFT, MP2) show that planar conformations reduce bandgap energy, while twisted backbones disrupt delocalization, affecting nonlinear optical (NLO) properties like hyperpolarizabilities. Experimental validation via second-harmonic generation (SHG) and electrochemical impedance spectroscopy quantifies these effects .
Q. What strategies resolve contradictions between theoretical and experimental electronic behavior?
- Hybrid DFT validation : Use B3LYP/6-31G* to reconcile computed torsional potentials with X-ray and UV data .
- Electrochemical benchmarking : Compare reduction potentials (e.g., cyclic voltammetry) of derivatives like 3,4'-dibromo-2,2'-bithiophene with theoretical predictions to identify discrepancies in electron affinity .
- Multi-scale modeling : Combine molecular dynamics (MD) with quantum mechanics (QM) to account for solvent and temperature effects in conformational equilibria .
Q. How does side-chain engineering (e.g., fluorination) enhance stability in this compound derivatives?
Fluorinated analogs (e.g., 3,4'-bis(1,1-difluoroalkyl)-2,2'-bithiophene) exhibit:
- Increased thermal stability : Fluorine's electronegativity strengthens C–F bonds, raising decomposition temperatures.
- Oxidative resistance : Fluorination reduces HOMO levels, minimizing undesired oxidation in organic electronics.
- Enhanced hydrophobicity : Improves environmental stability in polymer thin films. Synthesis involves Pd-catalyzed coupling of fluorinated alkyl halides with dibrominated precursors .
Q. How do molecular weight and alkyl chain length affect optoelectronic properties in polymers?
- Molecular weight : Higher degrees of polymerization (n > 20) reduce bandgap energy (via extended conjugation) but increase solubility challenges. Gel permeation chromatography (GPC) and UV-Vis track these trends .
- Alkyl chain optimization : Longer chains (e.g., hexyl vs. butyl) improve solubility without significantly altering HOMO-LUMO levels. Balancing side-chain length with π-conjugation is critical for organic field-effect transistors (OFETs) .
Q. Methodological Tables
Table 1. Key Computational Methods for Conformational Analysis
Method | Basis Set | Key Finding | Validation Technique |
---|---|---|---|
DFT (B3LYP) | 6-31G* | Syn-/anti-gauche minima | X-ray, UV-Vis |
MP2 | 6-31G* | Electron correlation effects | SHG measurements |
Table 2. Synthesis Yields for Derivatives
Derivative | Reaction Step | Yield (%) | Reference |
---|---|---|---|
3,4'-Dibromo-2,2'-bithiophene | Cross-coupling | 85 | |
This compound | Bromine-lithium exchange | 72 |
Properties
IUPAC Name |
3-hexyl-2-(4-hexylthiophen-2-yl)thiophene | |
---|---|---|
Source | PubChem | |
URL | https://pubchem.ncbi.nlm.nih.gov | |
Description | Data deposited in or computed by PubChem | |
InChI |
InChI=1S/C20H30S2/c1-3-5-7-9-11-17-15-19(22-16-17)20-18(13-14-21-20)12-10-8-6-4-2/h13-16H,3-12H2,1-2H3 | |
Source | PubChem | |
URL | https://pubchem.ncbi.nlm.nih.gov | |
Description | Data deposited in or computed by PubChem | |
InChI Key |
FWQMKAFKIYUBKB-UHFFFAOYSA-N | |
Source | PubChem | |
URL | https://pubchem.ncbi.nlm.nih.gov | |
Description | Data deposited in or computed by PubChem | |
Canonical SMILES |
CCCCCCC1=C(SC=C1)C2=CC(=CS2)CCCCCC | |
Source | PubChem | |
URL | https://pubchem.ncbi.nlm.nih.gov | |
Description | Data deposited in or computed by PubChem | |
Molecular Formula |
C20H30S2 | |
Source | PubChem | |
URL | https://pubchem.ncbi.nlm.nih.gov | |
Description | Data deposited in or computed by PubChem | |
DSSTOX Substance ID |
DTXSID10463167 | |
Record name | 3,4'-Dihexyl-2,2'-bithiophene | |
Source | EPA DSSTox | |
URL | https://comptox.epa.gov/dashboard/DTXSID10463167 | |
Description | DSSTox provides a high quality public chemistry resource for supporting improved predictive toxicology. | |
Molecular Weight |
334.6 g/mol | |
Source | PubChem | |
URL | https://pubchem.ncbi.nlm.nih.gov | |
Description | Data deposited in or computed by PubChem | |
CAS No. |
135926-93-1 | |
Record name | 3,4'-Dihexyl-2,2'-bithiophene | |
Source | EPA DSSTox | |
URL | https://comptox.epa.gov/dashboard/DTXSID10463167 | |
Description | DSSTox provides a high quality public chemistry resource for supporting improved predictive toxicology. | |
Retrosynthesis Analysis
AI-Powered Synthesis Planning: Our tool employs the Template_relevance Pistachio, Template_relevance Bkms_metabolic, Template_relevance Pistachio_ringbreaker, Template_relevance Reaxys, Template_relevance Reaxys_biocatalysis model, leveraging a vast database of chemical reactions to predict feasible synthetic routes.
One-Step Synthesis Focus: Specifically designed for one-step synthesis, it provides concise and direct routes for your target compounds, streamlining the synthesis process.
Accurate Predictions: Utilizing the extensive PISTACHIO, BKMS_METABOLIC, PISTACHIO_RINGBREAKER, REAXYS, REAXYS_BIOCATALYSIS database, our tool offers high-accuracy predictions, reflecting the latest in chemical research and data.
Strategy Settings
Precursor scoring | Relevance Heuristic |
---|---|
Min. plausibility | 0.01 |
Model | Template_relevance |
Template Set | Pistachio/Bkms_metabolic/Pistachio_ringbreaker/Reaxys/Reaxys_biocatalysis |
Top-N result to add to graph | 6 |
Feasible Synthetic Routes
Disclaimer and Information on In-Vitro Research Products
Please be aware that all articles and product information presented on BenchChem are intended solely for informational purposes. The products available for purchase on BenchChem are specifically designed for in-vitro studies, which are conducted outside of living organisms. In-vitro studies, derived from the Latin term "in glass," involve experiments performed in controlled laboratory settings using cells or tissues. It is important to note that these products are not categorized as medicines or drugs, and they have not received approval from the FDA for the prevention, treatment, or cure of any medical condition, ailment, or disease. We must emphasize that any form of bodily introduction of these products into humans or animals is strictly prohibited by law. It is essential to adhere to these guidelines to ensure compliance with legal and ethical standards in research and experimentation.