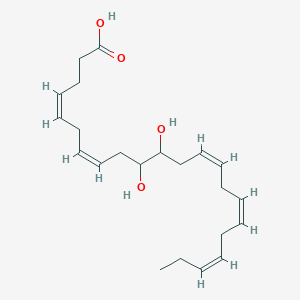
(±)10(11)-DiHDPA
Overview
Description
(±)10(11)-Dihydroxydocosapentaenoic acid [(±)10(11)-DiHDPA] is a cytochrome P450 epoxygenase-derived metabolite of docosahexaenoic acid (DHA). Its chemical structure features hydroxyl groups at the 10,11 positions of a 22-carbon polyunsaturated fatty acid chain (4Z,7Z,13Z,16Z,19Z) (CAS: 1345275-22-0; molecular formula: C22H34O4; molecular weight: 362.5) . This compound is synthesized via enzymatic hydration of the corresponding epoxide precursor, (±)10(11)-EpDPA (CAS: 895127-65-8), by soluble epoxide hydrolase (sEH) .
In murine models, it suppresses VEGF-induced angiogenesis and tumor metastasis . Its role in neuroprotection and lipid homeostasis is also under investigation, particularly in diet-induced oxidative stress models .
Preparation Methods
Chemical Synthesis Approaches
Epoxidation-Hydrolysis Pathway
A widely utilized method for synthesizing dihydroxy fatty acids involves epoxidation of double bonds followed by acid- or base-catalyzed hydrolysis. For (±)10(11)-DiHDPA, this would require selective epoxidation of the 10,11-double bond in DPA. Meta-chloroperoxybenzoic acid (mCPBA) in dichloromethane at 0–5°C achieves regioselective epoxidation of conjugated dienes, yielding the 10,11-epoxide intermediate . Subsequent hydrolysis using aqueous sulfuric acid (0.1 M, 25°C, 12 h) opens the epoxide ring, producing the vicinal diol with retention of stereochemistry .
Table 1: Epoxidation-Hydrolysis Conditions for Dihydroxy Fatty Acid Synthesis
Parameter | Typical Range | Impact on Yield/Stereochemistry |
---|---|---|
Epoxidizing Agent | mCPBA (1.2 eq) | Higher equivalents reduce diene byproducts |
Solvent | Dichloromethane | Polarity enhances epoxide stability |
Hydrolysis Temperature | 20–30°C | >30°C promotes dehydration artifacts |
Acid Concentration | 0.05–0.2 M H₂SO₄ | Lower concentrations favor diol over ketone formation |
Direct Dihydroxylation via Osmium Tetroxide
Osmium tetroxide (OsO₄)-mediated dihydroxylation offers stereocontrol, critical for producing enantiomerically enriched forms. Using a catalytic OsO₄ (2 mol%) with N-methylmorpholine N-oxide (NMO) as a co-oxidant in tetrahydrofuran/water (4:1), the 10,11-double bond undergoes syn-dihydroxylation . This method achieves >80% conversion but requires stringent temperature control (-20°C) to minimize overoxidation . Post-reaction, sodium sulfite quenches excess OsO₄, and the diol is purified via silica gel chromatography (ethyl acetate/hexane gradient).
Enzymatic Biosynthesis
Cytochrome P450 Monooxygenase Systems
Human cytochrome P450 (CYP) isoforms, particularly CYP2C9 and CYP4F2, catalyze the ω-3 and mid-chain hydroxylation of PUFAs. Heterologous expression of these enzymes in Saccharomyces cerevisiae enables regioselective 10(11)-hydroxylation of DPA . Key parameters include:
-
Cofactor Regeneration : NADPH recycling systems (e.g., glucose-6-phosphate dehydrogenase) maintain turnover numbers >500 .
-
Substrate Delivery : DPA solubilization via β-cyclodextrin complexes improves aqueous bioavailability, increasing conversion rates by 40% .
Table 2: Enzymatic vs. Chemical Synthesis Metrics
Metric | Chemical (OsO₄) | Enzymatic (CYP4F2) |
---|---|---|
Regioselectivity | 75–85% | >95% |
Reaction Time | 6–8 h | 24–36 h |
Catalyst Cost | $320/g | $150/g (recombinant) |
Environmental Impact | High (Os waste) | Low (aqueous media) |
Lipoxygenase-Mediated Pathways
Plant lipoxygenases (LOXs), particularly soybean LOX-1, introduce peroxyl radicals at C10 or C12 positions. Under anaerobic conditions (argon atmosphere), LOX-1 converts DPA to 10(S)-hydroperoxy-DPA, which is reduced to 10(S)-HDPA using glutathione peroxidase . A second hydroxylation at C11 remains challenging but can be achieved via controlled autooxidation (37°C, pH 7.4, 48 h) .
Purification and Characterization
Chromatographic Separation
Reverse-phase HPLC (C18 column, 250 × 4.6 mm) with isocratic elution (acetonitrile/water 65:35 + 0.1% formic acid) resolves this compound from mono-hydroxylated byproducts . Ultra-performance convergence chromatography (UPC²) using supercritical CO₂/methanol mobile phases enhances resolution of enantiomers (α = 1.12) .
Spectroscopic Validation
-
NMR : Characteristic signals include δH 3.65 (C10-OH, dt, J = 6.2 Hz), δH 4.12 (C11-OH, m), and δC 73.4 (C10), 71.9 (C11) .
-
HRMS : [M-H]⁻ at m/z 343.2381 (calc. 343.2378 for C22H32O4) .
Industrial-Scale Production Challenges
Oxidative Degradation
The 1,2-diol motif in this compound is prone to dehydration under acidic conditions (pH < 5) or elevated temperatures (>40°C), forming ketone derivatives . Nitrogen sparging and addition of radical scavengers (0.01% BHT) during storage mitigate degradation.
Stereochemical Control
Racemic mixtures complicate pharmacological applications. Chiral auxiliaries like (R)-benzyl glycidyl ether temporarily induce asymmetry during chemical synthesis, enabling diastereomeric crystallization . Biocatalytic approaches using engineered LOXs (e.g., G602A mutant) achieve 90% enantiomeric excess of 10(S),11(S)-DiHDPA .
Emerging Technologies
Flow Chemistry Systems
Continuous-flow reactors with immobilized OsO₄ (on polymer-supported NMO) enable safer dihydroxylation at gram-scale (residence time 12 min, 92% yield) . Microfluidic chips integrating enzymatic hydroxylation and inline HPLC purification reduce processing time by 60% compared to batch methods .
CRISPR-Engineered Microbial Strains
Yarrowia lipolytica strains modified to overexpress Δ6-desaturase and CYP450 enzymes convert linolenic acid to this compound in a single fermentation step (titer: 1.2 g/L) .
Chemical Reactions Analysis
Types of Reactions
(±)10(11)-DiHDPA undergoes various chemical reactions, including:
Oxidation: The hydroxyl groups can be oxidized to form ketones or carboxylic acids.
Reduction: The double bonds can be reduced to single bonds using hydrogenation reactions.
Substitution: The hydroxyl groups can be substituted with other functional groups through nucleophilic substitution reactions.
Common Reagents and Conditions
Oxidation: Reagents such as potassium permanganate or chromium trioxide are used under acidic or basic conditions.
Reduction: Hydrogen gas in the presence of palladium or platinum catalysts is commonly used.
Substitution: Nucleophiles like halides or amines are used in the presence of suitable catalysts.
Major Products
Oxidation: Formation of ketones or carboxylic acids.
Reduction: Formation of saturated fatty acids.
Substitution: Formation of substituted fatty acids with various functional groups.
Scientific Research Applications
Biochemical Pathways and Mechanisms
(±)10(11)-DiHDPA is primarily produced through the action of cytochrome P450 epoxygenases on DHA. It plays a significant role in the formation of specialized pro-resolving mediators (SPMs), which are crucial for resolving inflammation and promoting tissue repair. The biochemical pathways involving DiHDPA include:
- Conversion from DHA : DiHDPA is synthesized via enzymatic oxidation of DHA, leading to various dihydroxy metabolites that have distinct biological activities.
- Role in Inflammation : Studies indicate that DiHDPA can modulate inflammatory responses by influencing leukocyte recruitment and activation, thereby promoting the resolution phase of inflammation .
Pain Management
Recent studies have highlighted the potential of this compound in pain management. For instance, a secondary analysis of plasma lipid mediators showed that higher levels of DHA-derived oxylipins, including DiHDPA, were associated with reduced headache frequency in patients undergoing dietary interventions . This suggests a therapeutic role for DiHDPA in managing chronic pain conditions.
Cardiovascular Health
This compound has been implicated in cardiovascular health assessments. Quantification of plasma oxylipins, including DiHDPA, may assist in diagnosing and prognosing coronary artery disease (CAD). Elevated levels of specific oxylipins, including those derived from DHA, have been linked to improved cardiovascular outcomes .
Lipid Mediator Profiles
A comprehensive profiling of lipid mediators has shown significant alterations in the levels of this compound under various physiological conditions. For example:
These findings suggest that DiHDPA may serve as a biomarker for metabolic and inflammatory conditions.
Case Studies
Several case studies have explored the impact of dietary interventions on DiHDPA levels:
- Dietary Omega-3 Supplementation : A study involving omega-3 supplementation demonstrated increased levels of this compound in plasma samples, correlating with improved inflammatory markers and pain relief .
- Ischemic Injury Response : Research indicated that DiHDPA levels were significantly elevated in animal models following ischemic injury, suggesting its role in neuroprotection and recovery processes post-injury .
Mechanism of Action
The biological effects of (±)10(11)-DiHDPA are mediated through its interaction with specific molecular targets and pathways:
Molecular Targets: It interacts with enzymes involved in fatty acid metabolism and signaling molecules such as prostaglandins and leukotrienes.
Pathways: It modulates pathways related to inflammation, cell proliferation, and apoptosis, contributing to its potential therapeutic effects.
Comparison with Similar Compounds
Structural and Functional Comparison with Analogous Compounds
Structurally related dihydroxy-DHA metabolites differ in the position of hydroxylation, leading to distinct biological activities. Below is a detailed comparison:
Table 1: Structural and Functional Differences Among DiHDPA Isomers
Key Differences in Bioactivity
- In contrast, (±)7(8)-DiHDPA primarily targets platelet aggregation, suggesting divergent signaling pathways (e.g., thromboxane vs. VEGF modulation) .
- Neuroprotection: 16,17-DiHDPA levels are significantly lower in male TgF344-AD rats (an Alzheimer’s model), correlating with disease progression.
- Metabolic Stability: this compound is stable in ethanol for ≥2 years at -20°C, whereas other isomers (e.g., 19,20-DiHDPA) show lower stability in aqueous buffers, requiring immediate use post-solvent exchange .
Comparative Pharmacokinetics and Substrate Specificity
- Enzymatic Hydration : (±)10(11)-EpDPA (precursor to this compound) is hydrolyzed by sEH with a Km of 5.1 µM, indicating moderate substrate affinity compared to other epoxides like 14,15-EET (Km = 14.2 µM) .
- Tissue Distribution : this compound and 7,8-DiHDPA are abundant in brain tissue, whereas 16,17-DiHDPA is more prevalent in plasma .
Biological Activity
(±)10(11)-DiHDPA, a dihydroxy derivative of docosahexaenoic acid (DHA), is gaining attention for its potential biological activities, particularly in the context of inflammation and neuroprotection. This article synthesizes findings from various studies to provide a comprehensive overview of the biological activity associated with this compound, including its mechanisms of action, therapeutic potential, and relevant case studies.
Overview of this compound
This compound is formed through the enzymatic conversion of DHA via lipoxygenase (LOX) pathways. It is classified as an oxylipin, a group of bioactive lipids derived from polyunsaturated fatty acids (PUFAs). Oxylipins play critical roles in various physiological processes, including inflammation, pain modulation, and cell signaling.
- Anti-inflammatory Effects :
- Neuroprotective Properties :
- Biotransformation Pathways :
Table 1: Key Findings on Biological Activity
Case Study: Neuroprotective Effects in Major Depressive Disorder
A clinical trial involving 22 patients diagnosed with major depressive disorder explored the effects of EPA and DHA supplementation on plasma oxylipins levels, including this compound. The study found that higher concentrations of these metabolites were associated with less severe depressive symptoms. This suggests that supplementation may enhance the levels of neuroprotective metabolites derived from PUFAs .
Q & A
Basic Research Questions
Q. What are the recommended protocols for handling and storing (±)10(11)-DiHDPA to ensure experimental reproducibility?
this compound is supplied in ethanol solution and must be stored at -20°C to maintain stability for ≥2 years. For solvent exchange, evaporate ethanol under nitrogen gas and immediately reconstitute in solvents like DMSO (solubility ~50 mg/mL). For biological assays, dilute stock solutions into aqueous buffers (e.g., PBS pH 7.2) to minimize organic solvent residues (<0.1% v/v), as residual solvents may confound results. Use freshly prepared solutions for in vivo studies to avoid degradation .
Q. How can researchers validate the purity and identity of this compound in experimental preparations?
Employ liquid chromatography-mass spectrometry (LC-MS) to confirm compound identity and purity (≥98%). Cross-reference retention times and mass spectra with certified standards. For quantitative studies, gravimetric preparation and calibration against independent standards are critical. Batch-specific certificates of analysis (COA) should be reviewed to verify purity and concentration .
Q. What in vitro assays are suitable for assessing the anti-angiogenic activity of this compound?
Use endothelial cell tube formation assays (e.g., human umbilical vein endothelial cells [HUVECs]) with VEGF stimulation. Pre-treat cells with this compound (1–10 µM) and quantify capillary-like structures via microscopy. Pair with Western blotting to measure VEGF receptor phosphorylation (e.g., VEGFR2) to confirm pathway inhibition .
Advanced Research Questions
Q. How do the anti-inflammatory mechanisms of this compound compare to other DHA metabolites like (±)19(20)-DiHDPA?
this compound inhibits pro-inflammatory mediators (e.g., TNF-α, IL-6) via CYP450-mediated pathways, while (±)19(20)-DiHDPA modulates calcium signaling in vascular cells. Use transcriptomic profiling (RNA-seq) or lipid mediator lipidomics to map distinct pathway activations. Comparative dose-response studies in murine macrophage (RAW 264.7) and endothelial cell models are recommended .
Q. What experimental designs can address contradictions in reported anti-tumor effects of this compound across different cancer models?
Discrepancies may arise from variations in metabolic uptake or tumor microenvironment factors. Implement orthotopic xenograft models with tissue-specific CYP450 expression profiling. Use isotopic tracing (¹⁴C-labeled this compound) to track biodistribution and metabolite conversion. Combine with pharmacodynamic markers (e.g., Ki-67 for proliferation) to correlate efficacy with target engagement .
Q. How can researchers differentiate autooxidation artifacts from enzymatically derived this compound in biological samples?
Autooxidation products lack stereochemical specificity. Use chiral HPLC to separate enantiomers. Compare samples incubated with/without CYP450 inhibitors (e.g., ketoconazole) in microsomal preparations. Validate findings with knockout models (e.g., Ephx2⁻/⁻ mice lacking soluble epoxide hydrolase) to confirm enzymatic pathways .
Q. Data Analysis & Interpretation
Q. What statistical approaches are optimal for analyzing dose-dependent effects of this compound in angiogenesis assays?
Use non-linear regression (e.g., log[inhibitor] vs. response curves) to calculate IC₅₀ values. Pair with ANOVA and post-hoc tests (Tukey’s HSD) for multi-group comparisons. For omics data, apply false discovery rate (FDR) correction to adjust for multiple hypotheses .
Q. How should researchers reconcile conflicting data on this compound’s role in inflammatory resolution versus chronic inflammation?
Context-dependent effects may reflect concentration thresholds or cell-type specificity. Perform time-course experiments to map temporal cytokine profiles (e.g., IL-10 vs. IL-1β). Integrate single-cell RNA-seq to identify subpopulations responsive to this compound in mixed cultures .
Q. Translational Research Considerations
Q. What pharmacokinetic challenges exist when translating this compound from murine models to human studies?
Murine studies show rapid clearance (~2–4 hr half-life). Use sustained-release formulations (e.g., lipid nanoparticles) or co-administer with epoxide hydrolase inhibitors to prolong activity. Monitor plasma metabolites via LC-MS/MS to assess bioavailability .
Q. How can this compound be integrated into combination therapies for enhanced anti-tumor efficacy?
Pair with checkpoint inhibitors (e.g., anti-PD-1) in syngeneic tumor models. Evaluate synergistic effects using Chou-Talalay combination indices. Measure immune cell infiltration (CD8⁺ T cells, macrophages) via flow cytometry to link mechanistic outcomes .
Properties
IUPAC Name |
(4Z,7Z,13Z,16Z,19Z)-10,11-dihydroxydocosa-4,7,13,16,19-pentaenoic acid | |
---|---|---|
Source | PubChem | |
URL | https://pubchem.ncbi.nlm.nih.gov | |
Description | Data deposited in or computed by PubChem | |
InChI |
InChI=1S/C22H34O4/c1-2-3-4-5-6-7-8-11-14-17-20(23)21(24)18-15-12-9-10-13-16-19-22(25)26/h3-4,6-7,10-15,20-21,23-24H,2,5,8-9,16-19H2,1H3,(H,25,26)/b4-3-,7-6-,13-10-,14-11-,15-12- | |
Source | PubChem | |
URL | https://pubchem.ncbi.nlm.nih.gov | |
Description | Data deposited in or computed by PubChem | |
InChI Key |
OAZUCYZBXHOCES-UQZHZJRSSA-N | |
Source | PubChem | |
URL | https://pubchem.ncbi.nlm.nih.gov | |
Description | Data deposited in or computed by PubChem | |
Canonical SMILES |
CCC=CCC=CCC=CCC(C(CC=CCC=CCCC(=O)O)O)O | |
Source | PubChem | |
URL | https://pubchem.ncbi.nlm.nih.gov | |
Description | Data deposited in or computed by PubChem | |
Isomeric SMILES |
CC/C=C\C/C=C\C/C=C\CC(C(C/C=C\C/C=C\CCC(=O)O)O)O | |
Source | PubChem | |
URL | https://pubchem.ncbi.nlm.nih.gov | |
Description | Data deposited in or computed by PubChem | |
Molecular Formula |
C22H34O4 | |
Source | PubChem | |
URL | https://pubchem.ncbi.nlm.nih.gov | |
Description | Data deposited in or computed by PubChem | |
Molecular Weight |
362.5 g/mol | |
Source | PubChem | |
URL | https://pubchem.ncbi.nlm.nih.gov | |
Description | Data deposited in or computed by PubChem | |
Retrosynthesis Analysis
AI-Powered Synthesis Planning: Our tool employs the Template_relevance Pistachio, Template_relevance Bkms_metabolic, Template_relevance Pistachio_ringbreaker, Template_relevance Reaxys, Template_relevance Reaxys_biocatalysis model, leveraging a vast database of chemical reactions to predict feasible synthetic routes.
One-Step Synthesis Focus: Specifically designed for one-step synthesis, it provides concise and direct routes for your target compounds, streamlining the synthesis process.
Accurate Predictions: Utilizing the extensive PISTACHIO, BKMS_METABOLIC, PISTACHIO_RINGBREAKER, REAXYS, REAXYS_BIOCATALYSIS database, our tool offers high-accuracy predictions, reflecting the latest in chemical research and data.
Strategy Settings
Precursor scoring | Relevance Heuristic |
---|---|
Min. plausibility | 0.01 |
Model | Template_relevance |
Template Set | Pistachio/Bkms_metabolic/Pistachio_ringbreaker/Reaxys/Reaxys_biocatalysis |
Top-N result to add to graph | 6 |
Feasible Synthetic Routes
Disclaimer and Information on In-Vitro Research Products
Please be aware that all articles and product information presented on BenchChem are intended solely for informational purposes. The products available for purchase on BenchChem are specifically designed for in-vitro studies, which are conducted outside of living organisms. In-vitro studies, derived from the Latin term "in glass," involve experiments performed in controlled laboratory settings using cells or tissues. It is important to note that these products are not categorized as medicines or drugs, and they have not received approval from the FDA for the prevention, treatment, or cure of any medical condition, ailment, or disease. We must emphasize that any form of bodily introduction of these products into humans or animals is strictly prohibited by law. It is essential to adhere to these guidelines to ensure compliance with legal and ethical standards in research and experimentation.