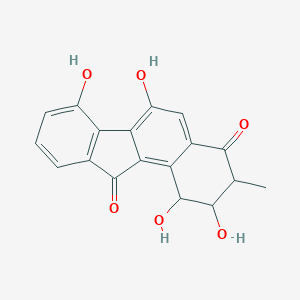
Fluostatin B
Overview
Description
Fluostatin B is a bioactive aromatic polyketide produced by Streptomyces species, notably Streptomyces sp. TA-3391 . Its molecular formula is C₁₈H₁₄O₆ (molecular weight: 326.30), featuring a benzofluorene core with two ketone groups (C-4 and C-11) and four hydroxyl groups (C-1, C-2, C-6, and C-7) . A key functional property is its inhibition of dipeptidyl peptidase III (DPP-III), with an IC₅₀ of 24.0 μg/mL using arginyl-arginine-2-naphthalene formamide as the substrate . This compound serves as a structural and biosynthetic reference for other members of the fluostatin family, which share a fluorone skeleton but vary in oxidation states, substituents, and ring systems .
Preparation Methods
Biosynthetic Origins and Type II Polyketide Synthase (PKS) Pathways
Fluostatin B is biosynthesized via a type II polyketide synthase (PKS) system, a modular enzymatic machinery responsible for assembling the polyketide backbone. In Streptomyces and Micromonospora species, the fls gene cluster orchestrates this process . The core PKS components—Fluo9, Fluo10, and Fluo11—catalyze the iterative condensation of one acetyl-CoA starter unit and nine malonyl-CoA extender units to form a linear poly-β-keto intermediate . This intermediate undergoes regioselective cyclization and aromatization mediated by cyclases (Fluo8 and Fluo13) and a ketoacyl reductase (Fluo12), yielding the angucycline scaffold .
A critical intermediate in this pathway is UWM6, which is further modified by a dehydratase (Fluo14) and an oxygenase (Fluo15) to produce dehydrorabelomycin . Subsequent enzymatic reductions and oxidations generate hydroquinone-kinobscurinone, a precursor to this compound . The stereochemical fidelity of these steps ensures the formation of the characteristic 6-5-6-6 ring system observed in this compound and related analogues .
Fermentation and Microbial Production
The production of this compound in microbial systems relies on optimized fermentation conditions. Streptomyces sp. PKU-MA00045 and Micromonospora rosaria SCSIO N160 are primary producers, with yields influenced by media composition, temperature, and aeration .
Table 1: Fermentation Parameters for this compound Production
Parameter | Optimal Condition | Impact on Yield |
---|---|---|
Carbon Source | Glycerol (2% w/v) | Enhances polyketide flux |
Nitrogen Source | Soybean meal (1.5% w/v) | Supports biomass growth |
Temperature | 28°C | Maximizes enzyme activity |
pH | 7.2–7.5 | Stabilizes PKS complexes |
Dissolved Oxygen | 30–40% saturation | Prevents anaerobic byproducts |
Extended fermentation periods (7–10 days) are required to achieve detectable titers of this compound, as the compound accumulates during stationary phase . Post-fermentation, extraction with ethyl acetate or methanol, followed by chromatographic purification (e.g., silica gel, HPLC), yields purified this compound .
Non-Enzymatic Dimerization and Post-Biosynthetic Modifications
A hallmark of this compound’s preparation is its propensity for non-enzymatic dimerization. Studies reveal that acylated fluostatin precursors undergo spontaneous deacylation in aqueous environments, generating reactive quinone methide intermediates . These intermediates facilitate C–C and C–N couplings, leading to dimeric products such as this compound .
For example, incubation of acyl fluostatin C (FST C) in water at 25°C produces this compound within 12 hours via a mechanism involving nucleophilic attack at the C-2 or C-3 positions . This process is pH-dependent, with optimal dimerization observed at neutral pH . The absence of enzymatic involvement in this step simplifies large-scale production, as it eliminates the need for specialized dimerization enzymes.
Genetic Engineering and Pathway Optimization
Genetic manipulation of the fls gene cluster has proven instrumental in enhancing this compound yields. Inactivation of flsN3, a gene encoding a glutamyl-tRNA amidotransferase, alters the biosynthetic pathway to favor this compound over N–N bond-containing analogues . Disruption of flsN3 in Micromonospora rosaria SCSIO N160 results in a 2.3-fold increase in this compound production, as confirmed by HPLC and NMR analyses .
Table 2: Key Genes in the this compound Biosynthetic Pathway
Additionally, heterologous expression of the fls cluster in Streptomyces albus J1074 has enabled scalable production, with titers reaching 120 mg/L under optimized conditions .
Purification and Analytical Characterization
Purification of this compound involves multi-step chromatographic methods. Crude extracts are subjected to silica gel column chromatography using a gradient of hexane/ethyl acetate (10:1 to 1:2), followed by reverse-phase HPLC (C18 column, acetonitrile/water) . Purity (>98%) is confirmed via LC-MS and -NMR spectroscopy .
Key spectroscopic features of this compound include:
Chemical Reactions Analysis
Types of Reactions: Fluostatin B undergoes various chemical reactions, including:
Oxidation: this compound can be oxidized to form more oxidized derivatives.
Reduction: Reduction reactions can modify the functional groups present in this compound.
Substitution: Substitution reactions can introduce new functional groups into the this compound molecule.
Common Reagents and Conditions:
Oxidation: Common oxidizing agents include potassium permanganate and chromium trioxide.
Reduction: Reducing agents such as sodium borohydride and lithium aluminum hydride are used.
Substitution: Substitution reactions often involve reagents like halogens or alkylating agents.
Major Products Formed: The major products formed from these reactions include various oxidized, reduced, and substituted derivatives of this compound, which can exhibit different biological activities.
Scientific Research Applications
Chemistry
Fluostatin B serves as a model compound for studying the biosynthesis and chemical properties of benzofluorene-containing angucyclines. Its unique structure allows researchers to explore the mechanisms of dimerization and the formation of complex compounds through π–π stacking interactions. This property is vital for understanding regio- and stereoselectivity in organic synthesis .
Biology
In biological research, this compound is used to investigate the inhibitory effects on enzymes, particularly DPP-3. The inhibition of this enzyme is significant as it plays a role in various physiological processes, including the regulation of peptide hormones. Studies have shown that this compound can inhibit other dipeptidyl peptidases, albeit to a lesser extent .
Case Study: Dipeptidyl Peptidase Inhibition
- Study : A comparative analysis of Fluostatin A and B revealed that while both compounds inhibit DPP-3, Fluostatin A has a lower IC50 value (0.44 µg/mL) compared to this compound (24 µg/mL) .
- Implication : This suggests potential for Fluostatin A in therapeutic applications targeting DPP-3 more effectively.
Medicine
This compound's inhibitory activity against DPP-3 positions it as a candidate for developing therapeutic agents aimed at conditions influenced by this enzyme. The modulation of DPP-3 activity could have implications in treating metabolic disorders and certain cancers .
Clinical Relevance
- Research Focus : Investigating statins' effects on cancer proliferation.
- Findings : In breast cancer studies, statins like Fluvastatin (related to this compound) have shown promising results in reducing tumor proliferation and increasing apoptosis in high-grade tumors .
Industry
In industrial applications, this compound and its derivatives are being explored for their potential in developing new pharmaceuticals and agrochemicals. The unique chemical structure of fluostatins lends itself to innovation in drug design and agricultural products .
Mechanism of Action
Fluostatin B is part of the fluostatin family, which includes several other compounds such as fluostatin A, fluostatin C, and fluostatin D. These compounds share a similar benzofluorene-containing angucycline structure but differ in their functional groups and biological activities . Compared to other fluostatins, this compound is unique due to its specific inhibitory activity against dipeptidyl peptidase 3 .
Comparison with Similar Compounds
Structural Analogues within the Fluostatin Family
Fluostatin C
- Molecular Formula : C₁₈H₁₄O₆ (identical to Fluostatin B) .
- Key Differences :
- Biosynthetic Relationship : Proposed as an oxidative derivative of this compound, sharing a common precursor with kinamycins .
Fluostatin D
- Molecular Formula : C₂₂H₂₀O₇ .
- Key Differences :
- Bioactivity : Similar to Fluostatin C but with enhanced lipophilicity due to the ester group .
Fluostatin E
Fluostatins M–Q
- Molecular Features :
- Structural Validation : X-ray diffraction confirmed the oxidized A-ring in Fluostatin P (4) .
- Biosynthesis : Gene cluster analysis revealed homology with kinamycin pathways, involving oxidases (AlpJ/AlpK) for ring contraction .
Comparative Data Table
Biological Activity
Fluostatin B is a member of the fluostatin family, which are aromatic polyketides produced by certain actinomycetes, notably Streptomyces and Micromonospora species. These compounds have garnered attention due to their diverse biological activities, particularly their potential as antitumor agents and inhibitors of specific enzymes. This article provides a comprehensive overview of the biological activity of this compound, including its mechanisms of action, pharmacological effects, and relevant case studies.
Chemical Structure and Biosynthesis
This compound features a complex structure characterized by a unique 6-5-6-6 ring system. Its biosynthesis involves a polyketide synthase (PKS) pathway, where various enzymes catalyze the formation of the compound from simple precursors. The gene clusters responsible for its biosynthesis have been identified in several Streptomyces strains, indicating a rich source for its production .
Biological Activity
1. Antitumor Activity
this compound exhibits notable antitumor properties. Preliminary pharmacological tests indicate that it inhibits the bioactivity of dipeptidyl peptidase III (DPP III), an enzyme involved in tumor progression. Additionally, this compound has shown moderate inhibitory effects against various human tumor cell lines, suggesting its potential as an anticancer agent .
2. Antibacterial Properties
Research has demonstrated that this compound possesses mild antibacterial activity against several bacterial strains. This activity is significant considering the increasing resistance to conventional antibiotics, positioning this compound as a candidate for further development in antimicrobial therapies .
3. Mechanisms of Action
The mechanisms underlying the biological activities of this compound are still being elucidated. However, studies suggest that its action may involve interference with key cellular pathways associated with tumor growth and bacterial survival. The non-enzymatic dimerization of fluostatins also plays a role in enhancing their bioactivity by forming more complex structures that may exhibit improved efficacy against target cells .
Table 1: Summary of Biological Activities of this compound
Case Study: In Vitro Effects on Tumor Cells
In one study, this compound was tested against several human cancer cell lines, including breast and colon cancer cells. The results indicated a dose-dependent inhibition of cell proliferation, with IC50 values suggesting significant potency compared to control treatments. The study concluded that further investigations into the molecular mechanisms are warranted to fully understand its therapeutic potential .
Case Study: Antimicrobial Efficacy
Another research effort focused on the antimicrobial properties of this compound against multi-drug resistant strains of Staphylococcus aureus. The compound demonstrated effective inhibition at relatively low concentrations, highlighting its potential as an alternative treatment option in the face of rising antibiotic resistance .
Q & A
Q. Basic: What experimental methods are recommended for synthesizing and characterizing Fluostatin B?
Methodological Answer:
Synthesis should follow protocols ensuring purity (>95%) via techniques like HPLC and NMR. Characterization requires spectral data (e.g., -NMR, -NMR, HRMS) and comparison with literature values. For novel derivatives, elemental analysis and X-ray crystallography may validate structural identity . Reproducibility is critical: document solvent systems, reaction temperatures, and catalyst ratios.
Q. Advanced: How can researchers resolve contradictions in reported bioactivity data for this compound?
Methodological Answer: Contradictions may arise from differences in assay conditions (e.g., cell lines, pH, incubation time). Address this by:
- Standardizing protocols (e.g., CLSI guidelines for antimicrobial assays).
- Conducting dose-response curves with internal controls.
- Performing meta-analyses to identify confounding variables (e.g., solvent polarity affecting solubility) .
- Validating findings using orthogonal assays (e.g., enzymatic inhibition vs. cell viability) .
Q. Basic: What in vitro models are suitable for assessing this compound’s bioactivity?
Methodological Answer:
Use cell lines relevant to the target pathway (e.g., cancer: HeLa, MCF-7; antimicrobial: S. aureus ATCC 25923). Include positive/negative controls and measure IC/MIC values. Statistical validation (e.g., ANOVA with post-hoc tests) ensures robustness .
Q. Advanced: How can mechanistic studies elucidate this compound’s mode of action?
Methodological Answer: Combine transcriptomics (RNA-seq) and proteomics (LC-MS/MS) to identify dysregulated pathways. CRISPR-Cas9 knockout models can validate target genes. Molecular docking (AutoDock Vina) and molecular dynamics simulations predict binding affinities to suspected targets (e.g., enzymes, receptors) .
Q. Basic: What statistical frameworks should guide experimental design for this compound studies?
Methodological Answer:
Adopt the PICOT framework (Population, Intervention, Comparison, Outcome, Time) to define scope. For hypothesis testing, use power analysis to determine sample size (G*Power software) and predefine significance thresholds (e.g., ) .
Q. Advanced: How can researchers apply the FINER criteria (Feasible, Interesting, Novel, Ethical, Relevant) to prioritize this compound research questions?
Methodological Answer:
- Feasibility: Assess resource availability (e.g., synthetic yield ≥50 mg).
- Novelty: Compare with existing derivatives in PubChem.
- Ethical: Follow IACUC protocols for animal studies.
- Relevance: Align with unmet therapeutic needs (e.g., antibiotic resistance) .
Q. Basic: How should stability studies for this compound be designed?
Methodological Answer:
Conduct accelerated stability testing under varied conditions (pH 2–9, 40°C/75% RH). Monitor degradation via LC-MS and quantify half-life using first-order kinetics. Report excipient compatibility if formulating .
Q. Advanced: What advanced analytical techniques validate this compound’s degradation products?
Methodological Answer: High-resolution mass spectrometry (HRMS) and -DOSY NMR differentiate degradation products. Computational tools (e.g., Schrödinger’s QikProp) predict metabolite toxicity .
Q. Basic: How to ensure reproducibility in this compound research?
Methodological Answer:
- Document synthetic steps in SI (e.g., molar ratios, purification methods).
- Share raw data (e.g., NMR spectra, assay readouts) in repositories like Zenodo.
- Adopt CONSORT guidelines for in vivo studies .
Q. Advanced: What strategies mitigate batch-to-batch variability in this compound production?
Methodological Answer: Implement quality-by-design (QbD) principles:
- Define critical quality attributes (CQAs: purity, solubility).
- Use design-of-experiments (DoE) to optimize reaction parameters.
- Apply PAT (Process Analytical Technology) for real-time monitoring .
Q. Basic: What ethical considerations apply to this compound animal studies?
Methodological Answer:
- Obtain IACUC approval (protocol # required).
- Use the ARRIVE 2.0 guidelines for reporting.
- Minimize sample size via power analysis .
Q. Advanced: How can multi-omics approaches enhance this compound’s translational potential?
Methodological Answer: Integrate metabolomics (untargeted LC-MS) and epigenomics (ChIP-seq) to map mechanism-of-action. Validate findings in patient-derived organoids .
Properties
CAS No. |
158906-40-2 |
---|---|
Molecular Formula |
C18H14O6 |
Molecular Weight |
326.3 g/mol |
IUPAC Name |
1,2,6,7-tetrahydroxy-3-methyl-2,3-dihydro-1H-benzo[a]fluorene-4,11-dione |
InChI |
InChI=1S/C18H14O6/c1-6-15(21)8-5-10(20)13-11-7(3-2-4-9(11)19)17(23)14(13)12(8)18(24)16(6)22/h2-6,16,18-20,22,24H,1H3 |
InChI Key |
PLKATXVLQJQTSA-UHFFFAOYSA-N |
SMILES |
CC1C(C(C2=C3C(=C(C=C2C1=O)O)C4=C(C3=O)C=CC=C4O)O)O |
Canonical SMILES |
CC1C(C(C2=C3C(=C(C=C2C1=O)O)C4=C(C3=O)C=CC=C4O)O)O |
Synonyms |
1,2,3,4-tetrahydro-1,2,6,7-tetrahydroxy-3-methyl-11H-benzo(a)fluorenone-4,11-dione fluostatin B |
Origin of Product |
United States |
Retrosynthesis Analysis
AI-Powered Synthesis Planning: Our tool employs the Template_relevance Pistachio, Template_relevance Bkms_metabolic, Template_relevance Pistachio_ringbreaker, Template_relevance Reaxys, Template_relevance Reaxys_biocatalysis model, leveraging a vast database of chemical reactions to predict feasible synthetic routes.
One-Step Synthesis Focus: Specifically designed for one-step synthesis, it provides concise and direct routes for your target compounds, streamlining the synthesis process.
Accurate Predictions: Utilizing the extensive PISTACHIO, BKMS_METABOLIC, PISTACHIO_RINGBREAKER, REAXYS, REAXYS_BIOCATALYSIS database, our tool offers high-accuracy predictions, reflecting the latest in chemical research and data.
Strategy Settings
Precursor scoring | Relevance Heuristic |
---|---|
Min. plausibility | 0.01 |
Model | Template_relevance |
Template Set | Pistachio/Bkms_metabolic/Pistachio_ringbreaker/Reaxys/Reaxys_biocatalysis |
Top-N result to add to graph | 6 |
Feasible Synthetic Routes
Disclaimer and Information on In-Vitro Research Products
Please be aware that all articles and product information presented on BenchChem are intended solely for informational purposes. The products available for purchase on BenchChem are specifically designed for in-vitro studies, which are conducted outside of living organisms. In-vitro studies, derived from the Latin term "in glass," involve experiments performed in controlled laboratory settings using cells or tissues. It is important to note that these products are not categorized as medicines or drugs, and they have not received approval from the FDA for the prevention, treatment, or cure of any medical condition, ailment, or disease. We must emphasize that any form of bodily introduction of these products into humans or animals is strictly prohibited by law. It is essential to adhere to these guidelines to ensure compliance with legal and ethical standards in research and experimentation.