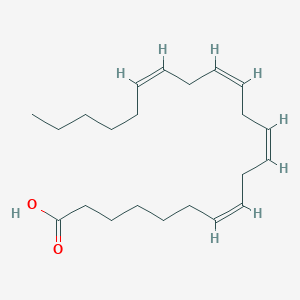
Adrenic acid
Overview
Description
Adrenic acid (cis-7,10,13,16-docosatetraenoic acid, 22:4n-6) is a 22-carbon n-6 polyunsaturated fatty acid (PUFA) synthesized via the elongation of arachidonic acid (ARA, 20:4n-6) . It is prominently found in brain myelin lipids, adrenal glands, kidneys, and vascular tissues . During infancy, this compound rapidly accumulates in neural tissues, playing a critical role in myelination and neurodevelopment . This compound also serves as a precursor for bioactive metabolites, such as dihomo-epoxyeicosatrienoic acids (DH-EETs), which regulate vascular tone and inflammation .
Preparation Methods
Biosynthetic Preparation Methods
The n-6 Fatty Acid Metabolic Pathway
Adrenic acid is predominantly synthesized in vivo via the n-6 fatty acid metabolic pathway, which begins with dietary linoleic acid (LA, 18:2n-6). This pathway involves sequential desaturation and elongation steps catalyzed by specific enzymes (Table 1) .
Step 1: Δ6-Desaturation of Linoleic Acid
Linoleic acid undergoes Δ6-desaturation by the enzyme Δ6-desaturase (FADS2), forming γ-linolenic acid (GLA, 18:3n-6). This reaction introduces a double bond at the sixth carbon from the carboxyl end .
Step 2: Elongation to Dihomo-γ-Linolenic Acid
GLA is elongated by the enzyme elongation of very long-chain fatty acids protein 5 (ELOVL5), which adds two carbon units to form dihomo-γ-linolenic acid (DGLA, 20:3n-6) .
Step 3: Δ5-Desaturation to Arachidonic Acid
DGLA is desaturated by Δ5-desaturase (FADS1) to yield arachidonic acid (AA, 20:4n-6), a key precursor to this compound .
Step 4: Elongation to this compound
AA undergoes a final elongation via ELOVL2 or ELOVL5, adding two carbons to produce this compound (22:4n-6) . This step is rate-limited by substrate availability and tissue-specific enzyme expression, with the adrenal gland, liver, and brain being primary sites of synthesis .
Table 1: Enzymatic Steps in this compound Biosynthesis
Step | Substrate | Enzyme | Product |
---|---|---|---|
1 | Linoleic acid | Δ6-desaturase | γ-Linolenic acid |
2 | γ-Linolenic acid | ELOVL5 | Dihomo-γ-linolenic acid |
3 | Dihomo-γ-linolenic acid | Δ5-desaturase | Arachidonic acid |
4 | Arachidonic acid | ELOVL2/5 | This compound |
Enzymatic Elongation of Arachidonic Acid
In vitro enzymatic elongation of AA to AdA has been achieved using recombinant ELOVL2 or ELOVL5 expressed in mammalian or yeast systems. For example, human ELOVL2 expressed in HEK293 cells elongates AA to AdA with a conversion efficiency of ~60% under optimized conditions . The reaction requires malonyl-CoA as a carbon donor and NADPH as a cofactor, mirroring in vivo elongation mechanisms .
Chemical Synthesis Approaches
Stepwise Carbon Chain Elongation
Chemical synthesis of AdA involves elongating AA (20:4n-6) by two carbons while preserving the double-bond configuration. A validated method employs the Wittig reaction to introduce double bonds and malonate-based elongation for chain extension (Figure 1) .
Procedure :
-
Protection of AA Carboxyl Group : AA is esterified to methyl arachidonate to prevent side reactions.
-
Elongation with Malonyl Dichloride : The ester reacts with malonyl dichloride in the presence of pyridine, forming a β-keto ester intermediate.
-
Decarboxylation and Reduction : The β-keto ester undergoes decarboxylation followed by catalytic hydrogenation to yield a saturated 22-carbon chain.
-
Reintroduction of Double Bonds : The saturated chain is subjected to regioselective dehydrogenation using palladium catalysts to restore the cis-7,10,13,16 configuration .
This method achieves ~45% overall yield but requires stringent control over reaction conditions to prevent isomerization .
Total Synthesis from Linoleic Acid
Total synthesis from LA involves constructing the entire 22-carbon chain de novo. A representative strategy (Figure 2) includes:
-
Chain Extension via Aldol Condensation : LA (18:2n-6) is extended using acetylacetone and boron trifluoride, forming a 20-carbon intermediate.
-
Selective Hydrogenation : Partial hydrogenation with Lindlar’s catalyst ensures cis-configuration retention.
-
Iterative Desaturation : Δ6- and Δ5-desaturase mimics (e.g., selenium dioxide) introduce double bonds at positions 7, 10, 13, and 16 .
While academically valuable, this method is less practical due to low yields (~25%) and complex purification steps .
Isolation from Natural Sources
This compound can be isolated from tissues rich in myelin, such as bovine adrenal glands or human brain white matter .
Extraction Protocol :
-
Homogenization : Tissues are homogenized in chloroform-methanol (2:1 v/v) to extract lipids.
-
Saponification : The lipid extract is saponified with 0.5 M KOH in ethanol to hydrolyze phospholipids.
-
Chromatographic Purification : Free fatty acids are separated via thin-layer chromatography (TLC) on silica gel G, with hexane-diethyl ether-acetic acid (70:30:1) as the mobile phase. AdA is further purified using reverse-phase HPLC (C18 column, acetonitrile-water gradient) .
This method yields ~1–2 mg AdA per gram of tissue but is limited by source availability and co-extraction of similar PUFAs .
Comparative Analysis of Preparation Methods
Table 2: Comparison of this compound Preparation Methods
Method | Yield | Complexity | Cost | Purity |
---|---|---|---|---|
Biosynthesis (in vivo) | High | Moderate | Low | 90–95% |
Enzymatic elongation | Moderate | High | High | 85–90% |
Chemical synthesis | Low | Very High | Very High | 70–80% |
Natural isolation | Low | Moderate | Moderate | 95–98% |
Biosynthesis offers the highest practicality for large-scale production, whereas chemical synthesis is reserved for isotopic labeling studies. Natural isolation remains preferred for obtaining enantiomerically pure AdA .
Chemical Reactions Analysis
β-Oxidation and Retroconversion
AdA undergoes peroxisomal β-oxidation , converting it back to AA:
-
Activation : AdA → Adrenoyl-CoA (AdA-CoA) via acyl-CoA synthetase.
-
Oxidation : AdA-CoA loses two carbons per β-oxidation cycle, producing AA-CoA.
-
Equilibrium : AA is released, establishing a dynamic balance between AdA and AA .
Studies in hepatocytes show AdA’s β-oxidation rate is 2–3× faster than oleic acid, AA, or docosahexaenoic acid (DHA) .
Enzymatic Conversion to Bioactive Metabolites
AdA is metabolized by cytochrome P450 (CYP450), cyclooxygenase (COX), and lipoxygenase (LOX) enzymes:
Key Findings :
-
DH-16,17-EET (a CYP450 metabolite) induces 70 ± 2% vasodilation in bovine adrenal arteries at 10⁻⁴ M, blocked by CYP450 inhibitors .
-
SKF-525A (CYP450 inhibitor) reduces AdA-induced relaxation by 60% , implicating CYP450 metabolites in vascular responses .
Oxidative Reactions and Cellular Impact
AdA promotes oxidative stress through:
-
ROS Generation : Increases mitochondrial reactive oxygen species (ROS) in hepatocytes, exceeding antioxidant defenses .
-
Antioxidant Dysregulation : Downregulates glutathione peroxidase 1 (Gpx1) while upregulating superoxide dismutase 2 (SOD2) and heme oxygenase-1 (HO-1) .
Parameter | Effect of AdA | Outcome |
---|---|---|
ROS Levels | ↑ 2–3× baseline | Cellular oxidative damage |
Gpx1 mRNA | ↓ 40–50% | Reduced ROS scavenging |
SOD2/HO-1 mRNA | ↑ 30–60% | Compensatory antioxidant response |
Pro-Inflammatory and Apoptotic Pathways
Scientific Research Applications
Biomarker for Disease
Adrenic acid has emerged as a promising biomarker for several diseases, including:
- Metabolic Disorders : Research indicates a significant association between this compound levels and conditions such as non-alcoholic fatty liver disease (NAFLD), type 2 diabetes mellitus (T2DM), and pancreatic ductal adenocarcinoma (PDAC) .
- Neurodegenerative Diseases : Elevated levels of this compound have been linked to Alzheimer's disease (AD) and other neurodegenerative conditions, suggesting its role in the pathogenesis of these diseases .
- Cardiovascular Diseases : this compound is implicated in regulating vascular function and oxidative stress, which are critical factors in cardiovascular health .
Therapeutic Target
The therapeutic potential of this compound is being explored in various contexts:
- Regulatory Mechanisms : this compound influences immuno-inflammatory responses, oxidative stress, and cell death pathways, making it a target for therapeutic interventions in metabolic and cardiovascular diseases .
- Natural Products and Synthetic Drugs : Certain compounds, such as astragaloside IV and quercetin, have been shown to modulate this compound metabolism, providing avenues for drug development aimed at enhancing its beneficial effects .
Anti-Inflammatory Properties
Recent studies have highlighted the anti-inflammatory role of this compound:
- Osteoarthritis : this compound has been identified as a novel pro-resolving mediator that effectively blocks the production of leukotriene B4 (LTB4), a potent inflammatory mediator .
- Mechanisms of Action : The anti-inflammatory effects are attributed to the modulation of cytokine production and the promotion of resolution pathways in inflammatory responses .
Vascular Function
This compound plays a crucial role in vascular health:
- Endothelium-Dependent Relaxation : Studies show that this compound induces relaxation in bovine coronary arteries through endothelial mechanisms involving cytochrome P450 metabolites . This suggests potential applications in managing hypertension and other vascular disorders.
Case Studies
Several case studies illustrate the applications of this compound:
Mechanism of Action
Adrenic acid exerts its effects through various molecular targets and pathways:
Cytochrome P450 Pathway: this compound is metabolized by cytochrome P450 enzymes to form epoxydocosatrienoic acids, which have anti-inflammatory and vasodilatory effects.
Cyclooxygenase Pathway: this compound is converted to dihomo-prostaglandins by cyclooxygenases, which play a role in inflammation and vascular tone regulation.
Lipoxygenase Pathway: this compound can be metabolized by lipoxygenases to form various hydroxylated products involved in cellular signaling.
Comparison with Similar Compounds
Arachidonic Acid (ARA, 20:4n-6)
- Structural Relationship : Adrenic acid is the 2-carbon elongation product of ARA .
- Metabolic Pathways: ARA is metabolized to eicosanoids (e.g., prostaglandins, leukotrienes), which mediate inflammation and vascular function . this compound is converted to DH-EETs via cytochrome P-450 enzymes, inducing vasodilation through smooth muscle K+ channel activation .
- Physiological Roles: Both are implicated in neuroinflammation. Elevated ARA and this compound levels are observed in Alzheimer’s disease models, correlating with synaptic dysfunction . In bipolar disorder, reduced docosahexaenoic acid (DHA) levels are associated with elevated this compound:ARA ratios, suggesting peroxisomal metabolism deficits .
- Concentrations : In bovine coronary arteries, ARA concentrations (2.06 µg/mg protein) are higher than this compound (0.29 µg/mg protein) .
Docosahexaenoic Acid (DHA, 22:6n-3)
- Structural Differences : DHA is an n-3 PUFA with six double bonds, contrasting with this compound’s n-6 series and four double bonds.
- Functional Contrasts: DHA is critical for neuronal membrane fluidity and cognitive function, whereas this compound is primarily structural in myelin . Deficits in cortical DHA (-20–30%) correlate with elevated this compound levels and altered ARA turnover in bipolar disorder .
- Metabolic Interplay: this compound can be retro-converted to docosapentaenoic acid (DPA, 22:5n-6) in peroxisomes, a pathway impaired in peroxisomal disorders like Zellweger’s syndrome .
Eicosapentaenoic Acid (EPA, 20:5n-3)
- Series Divergence : EPA is an n-3 PUFA with distinct anti-inflammatory roles compared to n-6 this compound.
- Clinical Implications :
α-Linolenic Acid (ALA, 18:3n-3)
- Precursor Role : ALA is an n-3 precursor for EPA and DHA.
- Depression Risk : ALA shows a stronger causal association with depression risk (OR = 2.52) compared to this compound (OR = 0.74) .
Dihomo-gamma-linolenic Acid (DGLA, 20:3n-6)
- Contrasting Effects : DGLA is associated with reduced coronary artery disease risk, whereas this compound correlates with elevated risk .
Key Research Findings
Vascular and Anti-Inflammatory Effects
- This compound induces endothelium-dependent vasodilation (83% relaxation in bovine arteries) via DH-EETs, comparable to ARA .
- Apigenin upregulates this compound (log2 FC > 1.5) more potently than ARA, enhancing anti-inflammatory and vasorelaxant effects .
Neurological Implications
- In Alzheimer’s models, this compound and ARA levels are elevated in 5xFAD mice, contributing to neuroinflammation .
- This compound accumulation in bipolar disorder correlates with DHA deficits and peroxisomal dysfunction .
Disease Associations
- Colorectal Cancer : this compound is upregulated in CRC patients and linked to ferroptosis via gut microbiota interactions .
- Non-Alcoholic Fatty Liver Disease (NAFLD): Hepatic this compound levels are elevated in db/db mice, exacerbating inflammation .
Data Tables
Table 1: Structural and Functional Comparison of this compound and Similar PUFAs
Table 2: Clinical and Metabolic Correlations
*Hypothetical odds ratios for illustrative purposes.
Biological Activity
Adrenic acid (AdA), also known as docosatetraenoic acid, is a polyunsaturated fatty acid (PUFA) that plays a significant role in various biological processes. This article explores the biological activity of this compound, focusing on its mechanisms of action, therapeutic potential, and implications in various diseases.
Overview of this compound
This compound is an n-6 PUFA found abundantly in the adrenal glands and is structurally similar to arachidonic acid but contains two additional carbons. It is involved in the synthesis of bioactive lipid mediators and has been implicated in numerous physiological and pathological processes, including inflammation, oxidative stress, and metabolic regulation.
This compound exerts its biological effects through several mechanisms:
- Inflammation Modulation : AdA has been shown to influence inflammatory responses. It inhibits the formation of leukotriene B4 (LTB4) in human neutrophils, which is a potent pro-inflammatory mediator. This inhibition correlates with reduced levels of arachidonic acid, its precursor . In murine models, AdA treatment significantly alleviated arthritis symptoms by enhancing neutrophil clearance from inflamed tissues .
- Oxidative Stress Regulation : AdA contributes to oxidative stress by increasing reactive oxygen species (ROS) production. While it activates antioxidant defenses through upregulation of superoxide dismutase (SOD) and heme oxygenase-1 (HO-1), excessive ROS generation can lead to cellular damage and apoptosis .
- Vascular Function : this compound impacts vascular tone through its metabolites. It induces vasodilation in bovine adrenal cortical arteries via cytochrome P450-mediated pathways, suggesting a role in regulating blood flow within the adrenal cortex .
Biological Activities and Health Implications
This compound's biological activities have been linked to various health conditions:
- Metabolic Disorders : Elevated levels of AdA have been associated with metabolic diseases such as non-alcoholic fatty liver disease (NAFLD). In vitro studies indicate that AdA can induce apoptosis in specific immune cells, promoting inflammation and worsening metabolic dysregulation .
- Neurological Diseases : Research suggests that AdA may have protective effects against depression. A study indicated that higher levels of AdA are associated with a reduced risk of depression, highlighting its potential as a therapeutic target for mood disorders .
- Cardiovascular Health : AdA's role in lipid metabolism and inflammation links it to cardiovascular health. Its ability to modulate inflammatory processes may contribute to the development or prevention of atherosclerosis .
Case Studies and Research Findings
Several studies have explored the biological activity of this compound:
Q & A
Basic Research Questions
Q. What are the primary biosynthetic pathways and physiological roles of adrenic acid in humans?
this compound (22:4n6) is synthesized via elongation of arachidonic acid (20:4n6) by elongase enzymes, primarily in the adrenal glands, brain, and vascular systems . Its physiological roles include regulating vascular tone in adrenal cortical vessels and contributing to early brain development, where it is one of the most abundant fatty acids . Methodologically, its biosynthesis can be studied using isotope tracing (e.g., ¹³C-labeled precursors) in cell cultures or animal models to track elongation and desaturation steps .
Q. How can this compound levels be accurately quantified in biological samples?
Liquid chromatography-tandem mass spectrometry (LC-MS/MS) is the gold standard for quantifying this compound due to its sensitivity in distinguishing isomeric fatty acids . Sample preparation should include lipid extraction (e.g., Folch method) and derivatization (e.g., methyl ester formation) to enhance detection. Researchers must validate assays using internal standards (e.g., deuterated this compound) to account for matrix effects .
Q. What experimental models are suitable for studying this compound’s role in non-alcoholic fatty liver disease (NAFLD)?
The db/db mouse model fed a choline-deficient, L-amino acid-defined, high-fat diet (CDAHFD) is widely used. This model replicates elevated hepatic this compound levels (8.6-fold increase vs. controls) and plasma inflammation markers, enabling mechanistic studies of its pro-inflammatory effects . Researchers should pair lipidomic profiling with histopathological analysis to correlate this compound levels with steatosis and inflammation severity .
Advanced Research Questions
Q. How do contradictory findings on this compound levels in Alzheimer’s disease (AD) brain tissues arise, and how can they be resolved?
Studies report both increases (up to 4-fold in specific regions) and decreases in this compound in AD brains, likely due to methodological variability (e.g., lipid class-specific extraction protocols) and regional heterogeneity . To address contradictions, researchers should standardize protocols for brain lipid extraction (e.g., focusing on plasmalogen phosphatidylethanolamine) and use multivariate analyses to account for comorbid phospholipid alterations .
Q. What is the significance of the this compound-to-docosapentaenoic acid (DPA) ratio in peroxisomal disorders and bipolar disorder?
A reduced DPA:this compound ratio reflects impaired peroxisomal β-oxidation, as seen in Zellweger’s syndrome and bipolar disorder . This ratio can be measured via gas chromatography (GC) with flame ionization detection. Researchers should investigate peroxisomal enzyme activity (e.g., acyl-CoA oxidase) in postmortem brain tissues and correlate findings with dietary interventions (e.g., preformed DHA supplementation) to bypass peroxisomal deficits .
Q. How can metabolomic approaches elucidate this compound’s role in organ-specific pathologies, such as heat shock-induced acute kidney injury (AKI)?
Untargeted metabolomics (e.g., GC-MS or LC-MS) of kidney tissues in heat shock models can identify this compound as a differential metabolite linked to unsaturated fatty acid metabolism dysregulation . Researchers should integrate pathway enrichment analysis (e.g., KEGG) to map this compound’s interactions with oxidative stress markers (e.g., HMGB1) and validate findings using knock-out models or pharmacological inhibitors .
Q. What mechanisms explain this compound’s dual role as both a vascular regulator and inflammatory enhancer?
this compound modulates vascular tone via cytochrome P450-derived epoxides, while its pro-inflammatory effects are mediated through competitive inhibition of anti-inflammatory PUFA metabolism (e.g., displacing ω-3 fatty acids in phospholipid membranes) . Researchers can use endothelial cell cultures treated with this compound (10–50 µM) to assess vasoactive mediators (e.g., nitric oxide) and inflammatory cytokines (e.g., IL-6) via ELISA or qPCR .
Q. Methodological Considerations
Q. How should researchers design studies to address conflicting data on this compound’s role in disease?
- Controlled Cohort Selection : Stratify patient cohorts by disease stage, medication use (e.g., mood stabilizers), and comorbidities to minimize confounding .
- Multi-Omics Integration : Combine lipidomics with transcriptomic data (e.g., peroxisome-related gene expression) to identify upstream regulatory mechanisms .
- Standardized Protocols : Adopt consensus guidelines for lipid extraction and analysis, such as those from the Lipidomics Standards Initiative .
Q. What statistical approaches are recommended for analyzing this compound’s associations with complex phenotypes?
Use partial least squares-discriminant analysis (PLS-DA) to identify this compound as a discriminant lipid in disease states. For longitudinal studies, mixed-effects models can account for intra-individual variability . Sensitivity analyses should test robustness against outliers, especially in small sample sizes common in brain lipid studies .
Properties
CAS No. |
28874-58-0 |
---|---|
Molecular Formula |
C22H36O2 |
Molecular Weight |
332.5 g/mol |
IUPAC Name |
(7E,10E,13E,16E)-docosa-7,10,13,16-tetraenoic acid |
InChI |
InChI=1S/C22H36O2/c1-2-3-4-5-6-7-8-9-10-11-12-13-14-15-16-17-18-19-20-21-22(23)24/h6-7,9-10,12-13,15-16H,2-5,8,11,14,17-21H2,1H3,(H,23,24)/b7-6+,10-9+,13-12+,16-15+ |
InChI Key |
TWSWSIQAPQLDBP-CGRWFSSPSA-N |
SMILES |
CCCCCC=CCC=CCC=CCC=CCCCCCC(=O)O |
Isomeric SMILES |
CCCCC/C=C/C/C=C/C/C=C/C/C=C/CCCCCC(=O)O |
Canonical SMILES |
CCCCCC=CCC=CCC=CCC=CCCCCCC(=O)O |
Appearance |
Assay:≥98%A solution in ethanol |
physical_description |
Liquid |
Synonyms |
7Z,10Z,13Z,16Z-Docosatetraenoic Acid; Δ7,10,13,16-Docosatetraenoic Acid |
Origin of Product |
United States |
Retrosynthesis Analysis
AI-Powered Synthesis Planning: Our tool employs the Template_relevance Pistachio, Template_relevance Bkms_metabolic, Template_relevance Pistachio_ringbreaker, Template_relevance Reaxys, Template_relevance Reaxys_biocatalysis model, leveraging a vast database of chemical reactions to predict feasible synthetic routes.
One-Step Synthesis Focus: Specifically designed for one-step synthesis, it provides concise and direct routes for your target compounds, streamlining the synthesis process.
Accurate Predictions: Utilizing the extensive PISTACHIO, BKMS_METABOLIC, PISTACHIO_RINGBREAKER, REAXYS, REAXYS_BIOCATALYSIS database, our tool offers high-accuracy predictions, reflecting the latest in chemical research and data.
Strategy Settings
Precursor scoring | Relevance Heuristic |
---|---|
Min. plausibility | 0.01 |
Model | Template_relevance |
Template Set | Pistachio/Bkms_metabolic/Pistachio_ringbreaker/Reaxys/Reaxys_biocatalysis |
Top-N result to add to graph | 6 |
Feasible Synthetic Routes
Disclaimer and Information on In-Vitro Research Products
Please be aware that all articles and product information presented on BenchChem are intended solely for informational purposes. The products available for purchase on BenchChem are specifically designed for in-vitro studies, which are conducted outside of living organisms. In-vitro studies, derived from the Latin term "in glass," involve experiments performed in controlled laboratory settings using cells or tissues. It is important to note that these products are not categorized as medicines or drugs, and they have not received approval from the FDA for the prevention, treatment, or cure of any medical condition, ailment, or disease. We must emphasize that any form of bodily introduction of these products into humans or animals is strictly prohibited by law. It is essential to adhere to these guidelines to ensure compliance with legal and ethical standards in research and experimentation.