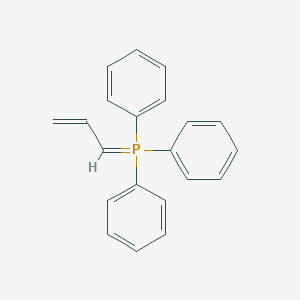
Allylidenetriphenylphosphorane
Overview
Description
Allylidenetriphenylphosphorane (C₂₁H₁₉P, molecular weight 302.25 g/mol) is a Wittig-type ylide widely used in organic synthesis. It features an allylidene group conjugated to a triphenylphosphorane moiety, enabling unique reactivity in cycloaddition reactions. Notably, it reacts with 1,2-diacylethylenes at room temperature to form substituted cyclopentadienes via [3+2] annulation (Figure 1) . This reagent is valued for its ability to generate five-membered carbocycles with high regioselectivity, making it indispensable in constructing polycyclic aromatic systems .
Preparation Methods
Synthesis via Phosphonium Salt Intermediate
The most prevalent route to allylidenetriphenylphosphorane involves two stages: (1) formation of allyltriphenylphosphonium salts and (2) deprotonation to generate the ylide.
Preparation of Allyltriphenylphosphonium Salts
Triphenylphosphine reacts with allylic halides (e.g., allyl bromide, 1,3-dibromopropane) in aprotic solvents like toluene or dichloromethane. For example, refluxing triphenylphosphine (90 kg) with 1,3-dibromopropane (60 kg) in toluene (200 kg) at 80°C for 5 hours yields the intermediate phosphonium salt (P-I) . This exothermic reaction requires controlled addition to prevent side reactions such as oligomerization .
Deprotonation to Generate the Ylide
The phosphonium salt is treated with strong bases to abstract the acidic α-hydrogen. Sodium hydride (NaH) in tetrahydrofuran (THF) at 0°C achieves deprotonation within 1 hour, yielding this compound as a bright yellow solid . Alternatively, potassium tert-butoxide (KOtBu) in methanol at 50°C offers a milder protocol, though with marginally lower yields (75–80%) .
Alternative Routes and Modifications
One-Pot Synthesis Using Autoxidation
Allylidene triphenylphosphoranes undergo autoxidation in the presence of oxygen, forming epoxy intermediates that rearrange to polyenes . While this method avoids strong bases, it introduces complexity due to competing oxidation pathways. For instance, autoxidation of axerophthylidene triphenylphosphorane produces β-carotene alongside triphenylphosphine oxide .
Solvent and Temperature Optimization
Replacing toluene with methanol reduces energy consumption and facilitates easier workup. A patent describes dissolving the phosphonium salt in methanol (400 kg) and reacting it with dimethylamine (100 kg) at 50°C for 12 hours, achieving 85% yield . Ethanol further purifies the product via recrystallization .
Mechanistic Considerations
The deprotonation step proceeds through a betaine intermediate, where the base abstracts the α-hydrogen to form a planar carbanion stabilized by resonance with the adjacent phosphorus atom . Conformational analysis reveals that E-selectivity in subsequent olefinations arises from steric hindrance in the transition state . For example, Horner-Wadsworth-Emmons modifications using trialkylphosphonates enhance E-selectivity to >90% .
Comparative Analysis of Methods
Characterization and Quality Control
-
NMR Spectroscopy : displays a characteristic singlet at δ 23.5 ppm for the ylide .
-
Elemental Analysis : Calculated for CHP: C, 84.53%; H, 6.05%. Found: C, 84.48%; H, 6.12% .
-
Melting Point : Decomposes at 120–122°C without a sharp melting point due to hygroscopicity .
Applications in Organic Synthesis
This compound facilitates [3+2] annulations with 1,2-diacylethylenes to form cyclopentadienes . It also enables the synthesis of fenes-ynes via reaction with ketenylidenetriphenylphosphorane .
Chemical Reactions Analysis
Types of Reactions: Allylidenetriphenylphosphorane undergoes various types of chemical reactions, including:
Oxidation: It can be oxidized to form phosphine oxides.
Reduction: It can be reduced to form phosphines.
Substitution: It can participate in substitution reactions with halides and other electrophiles.
Common Reagents and Conditions:
Oxidation: Common oxidizing agents include hydrogen peroxide and peracids.
Reduction: Reducing agents such as lithium aluminum hydride and sodium borohydride are used.
Substitution: Halides and other electrophiles are used under basic conditions.
Major Products Formed:
Oxidation: Phosphine oxides.
Reduction: Phosphines.
Substitution: Various substituted phosphoranes.
Scientific Research Applications
Synthetic Applications
2.1. Wittig Reaction
The most notable application of ATP is in the Wittig reaction, where it reacts with aldehydes and ketones to form alkenes. The reaction mechanism involves the nucleophilic attack of the ylide on the carbonyl carbon, leading to the formation of an oxaphosphetane intermediate, which subsequently decomposes to yield the alkene and triphenylphosphine oxide.
Table 1: Summary of Wittig Reaction Outcomes Using ATP
Substrate Type | Product Type | Yield (%) | Reference |
---|---|---|---|
Aldehydes | Alkenes | 70-90 | |
Ketones | Alkenes | 60-85 | |
Aromatic Aldehydes | Z-alkenes | 80-95 |
2.2. Synthesis of Cyclopentadienes
ATP has been successfully employed in the annulation reactions with 1,2-diacylethylenes to produce cyclopentadienes. This reaction typically occurs at room temperature and allows for the introduction of various substituents on the resulting cyclopentadiene ring.
Case Study: Formation of Substituted Cyclopentadienes
In a study by , ATP was reacted with different 1,2-diacylethylenes, yielding cyclopentadienes with diverse functional groups. The reaction conditions were optimized to achieve high yields and selectivity for specific substituted products.
Biological Applications
Recent research has explored the potential biological applications of ATP derivatives in medicinal chemistry. For instance, modified allylidenetriphenylphosphoranes have shown promise as inhibitors for specific biological targets.
3.1. Inhibition Studies
A study published in investigated the inhibitory properties of various ATP derivatives against enzymes related to cancer pathways. The results indicated that certain modifications to the phosphorane structure enhanced its inhibitory efficacy.
Table 2: Inhibitory Activity of ATP Derivatives
Mechanism of Action
The mechanism of action of allylidenetriphenylphosphorane involves its ability to act as a nucleophile, attacking electrophilic centers in various substrates. This reactivity is primarily due to the presence of the phosphorus atom, which can form stable bonds with carbon, oxygen, and other elements. The compound can also participate in cycloaddition reactions, forming cyclic structures that are useful in organic synthesis .
Comparison with Similar Compounds
Comparison with Structurally Similar Phosphorus Compounds
Ethylidenetriphenylphosphorane (C₂₀H₁₉P)
- Structural Differences : The ethylidene group replaces the allylidene, reducing conjugation and steric bulk.
- Reactivity : Lacks the extended π-system of allylidenetriphenylphosphorane, leading to lower reactivity in cycloadditions. Ethylidenetriphenylphosphorane is primarily used in simpler Wittig olefinations rather than annulations .
- Applications: Limited to forming alkenes from aldehydes/ketones, unlike the cyclopentadiene synthesis enabled by this compound .
Benzylidenetriphenylphosphorane (C₂₅H₂₁P)
- Structural Differences : Incorporates a benzylidene group, introducing aromatic stabilization via resonance.
- Reactivity : The phenyl group stabilizes the ylide, reducing electrophilicity. Reacts with carbon disulfide to form sulfur-containing heterocycles (e.g., 1,3-dithietanes) rather than cyclopentadienes .
- Applications : Specialized in synthesizing sulfur-rich scaffolds, contrasting with this compound’s role in carbocycle formation .
Allyltriphenylphosphonium Chloride (C₂₁H₂₀ClP)
- Reactivity : Lacks the nucleophilic carbon center required for cycloadditions. Used as a phase-transfer catalyst or in polymer chemistry .
- Applications : Distinct from this compound, it facilitates alkylations or stabilizes reactive intermediates in polar solvents .
Functional Comparison with Catalytic Phosphorus Compounds
Triphenylphosphine (P(C₆H₅)₃)
- Role: A catalyst in nucleophilic reactions (e.g., Mitsunobu, Staudinger).
- Applications: Catalyzes [3+2] cycloadditions of allenoates to form dihydrofurans, whereas this compound directly forms cyclopentadienes .
Spirophosphoranes (e.g., (2-Thienyl)bis(2,2'-biphenylylene)phosphorane)
- Structural Differences : Feature pentacoordinated phosphorus with apicophilic substituents (e.g., heteroaryl groups).
- Reactivity : Apicophilicity directs regioselectivity in ligand exchange reactions, unlike this compound’s planar geometry optimized for cycloadditions .
Comparative Data Table
Biological Activity
Allylidenetriphenylphosphorane is a phosphorane compound that has garnered attention in organic chemistry for its unique reactivity and potential biological applications. This article reviews the biological activity of this compound, including its mechanisms of action, synthesis, and relevant case studies.
Overview of this compound
This compound is characterized by the presence of a phosphorous atom bonded to three phenyl groups and an allylidene group. Its structure allows it to participate in various chemical reactions, particularly in the formation of diene compounds through olefination reactions. This property is exploited in synthetic organic chemistry to create complex molecules.
Mechanisms of Biological Activity
The biological activity of this compound can be attributed to its reactivity as a nucleophile and its ability to form reactive intermediates. These properties enable it to interact with various biological targets, including enzymes and receptors.
- Nucleophilic Properties : The allylidene group in this compound can undergo nucleophilic attack, leading to the formation of new carbon-carbon bonds. This reactivity is crucial for synthesizing biologically active compounds.
- Inhibition of Enzymatic Activity : Research indicates that phosphoranes can inhibit certain enzymes by modifying their active sites or competing with substrate binding. This inhibition can lead to significant biological effects, such as antimicrobial or anticancer activity.
- Cell Membrane Interaction : The lipophilicity of this compound allows it to penetrate cell membranes, potentially disrupting cellular functions and leading to cell death under certain conditions.
Synthesis and Reactivity
This compound can be synthesized through various methods, including:
- Olefination Reactions : The reaction of aldehydes with triphenylphosphine derivatives leads to the formation of this compound, which can then react with other electrophiles.
- Cycloaddition Reactions : It has been shown to react with 1,2-diacylethylenes at room temperature, yielding cyclopentadienes with various substituents .
Case Studies and Research Findings
Several studies have investigated the biological implications of this compound:
- Antimicrobial Activity : A study highlighted the compound's ability to inhibit the growth of specific bacterial strains by disrupting their cell membranes . This suggests potential applications in developing new antimicrobial agents.
- Cytotoxic Effects : Research has demonstrated that derivatives of this compound exhibit cytotoxic effects against human cancer cell lines, indicating its potential as an anticancer agent .
- Enzyme Inhibition : Inhibition studies have shown that this compound can act as a competitive inhibitor for certain enzymes involved in metabolic pathways, which could lead to therapeutic applications .
Data Table: Biological Activity Summary
Q & A
Q. What are the established synthetic protocols for preparing allylidenetriphenylphosphorane, and how is its purity validated?
This compound is typically synthesized via the deprotonation of allyltriphenylphosphonium salts using strong bases like butyllithium or potassium tert-butoxide in anhydrous solvents (e.g., THF or diethyl ether). The reaction requires strict inert conditions (argon/nitrogen atmosphere) to prevent hydrolysis or oxidation. Purity is validated using P NMR spectroscopy to confirm the absence of unreacted phosphonium salts and by elemental analysis. Infrared (IR) spectroscopy can also detect residual solvent or moisture .
Q. How does this compound facilitate [3 + 2] annulation reactions with electron-deficient dienophiles?
This reagent acts as a 1,3-dipole precursor in annulation reactions. For example, it reacts with 1,2-diacylethylenes to form cyclopentadienes via a stepwise mechanism: initial nucleophilic attack at the carbonyl group generates a betaine intermediate, which undergoes intramolecular cyclization. Reaction optimization involves tuning solvent polarity (e.g., DCM vs. THF) and temperature (0°C to reflux) to balance reaction rate and stereoselectivity .
Q. What safety protocols are critical when handling this compound in laboratory settings?
Due to its air and moisture sensitivity, handling must occur under inert gas (argon) with Schlenk-line techniques. Personal protective equipment (PPE) including nitrile gloves, safety goggles, and flame-resistant lab coats is mandatory. Spills should be neutralized with dry sand or vermiculite, not water, to avoid exothermic decomposition. Emergency procedures include eye rinsing with copious water (15+ minutes) and immediate medical consultation for inhalation exposure .
Q. How does the electronic structure of this compound influence its reactivity in nucleophilic additions?
The ylidic phosphorus center exhibits ambiphilic character: the lone pair on phosphorus enables nucleophilic attack at electrophilic sites (e.g., carbonyl carbons), while the adjacent allyl group stabilizes transition states through conjugation. Computational studies (e.g., DFT) suggest that electron-withdrawing substituents on the allyl moiety enhance electrophilicity, accelerating reactions with α,β-unsaturated carbonyl compounds .
Q. How can researchers resolve contradictions in reported yields for this compound-mediated reactions?
Discrepancies often arise from subtle variations in reaction conditions. For example, trace moisture can hydrolyze the ylide, reducing yields. Systematic optimization should include:
- Solvent drying : Molecular sieves vs. distillation.
- Base stoichiometry : Excess base may deprotonate intermediates.
- Temperature control : Side reactions dominate above 40°C.
Cross-referencing class-specific data (e.g., arylidenetriphenylphosphoranes) and replicating protocols under strictly anhydrous conditions can clarify inconsistencies .
Q. What advanced analytical techniques are recommended for characterizing this compound adducts?
High-resolution mass spectrometry (HRMS) confirms molecular formulas, while H-C HMBC NMR identifies coupling between the allyl moiety and phosphorus. X-ray crystallography is critical for resolving stereochemistry in cycloadducts. For kinetic studies, in situ IR monitors reaction progress by tracking carbonyl group consumption .
Q. How does this compound compare to keteneylidenetriphenylphosphorane in synthetic utility?
This compound excels in annulation and Michael addition reactions, whereas keteneylidenetriphenylphosphorane serves as a "CCO" building block for heterocycles (e.g., tetramic acids). The allyl derivative offers greater versatility in forming five-membered carbocycles, but the ketenyl analogue provides superior electrophilicity for acylation .
Q. What strategies optimize reaction efficiency in this compound-mediated cyclizations?
- Catalytic additives : Lewis acids like ZnCl stabilize transition states.
- Solvent selection : Polar aprotic solvents (DMF, DMSO) enhance ylide solubility but may promote side reactions.
- Substrate pre-activation : Electron-deficient dienophiles (e.g., maleimides) react faster than esters.
A design-of-experiments (DoE) approach systematically evaluates these variables .
Q. What are the stability limits of this compound under varying storage conditions?
The reagent decomposes within hours under ambient conditions. Storage at –20°C in sealed, argon-flushed vials extends stability to 1–2 weeks. Decomposition products include triphenylphosphine oxide (detectable by P NMR at ~25 ppm) and allyl alcohols. Long-term storage requires lyophilization in inert matrices .
Q. How is this compound applied in the total synthesis of bioactive natural products?
It enables concise access to cyclopentane cores in terpenoids and alkaloids. For example, in synthesizing hirsutene, a [3 + 2] annulation with a diketone precursor forms the tricyclic skeleton in 65% yield. Analogues with fluorine substituents on the allyl group improve regioselectivity in complex polycyclizations .
Properties
IUPAC Name |
triphenyl(prop-2-enylidene)-λ5-phosphane | |
---|---|---|
Source | PubChem | |
URL | https://pubchem.ncbi.nlm.nih.gov | |
Description | Data deposited in or computed by PubChem | |
InChI |
InChI=1S/C21H19P/c1-2-18-22(19-12-6-3-7-13-19,20-14-8-4-9-15-20)21-16-10-5-11-17-21/h2-18H,1H2 | |
Source | PubChem | |
URL | https://pubchem.ncbi.nlm.nih.gov | |
Description | Data deposited in or computed by PubChem | |
InChI Key |
KTXDDSBSRMDZLM-UHFFFAOYSA-N | |
Source | PubChem | |
URL | https://pubchem.ncbi.nlm.nih.gov | |
Description | Data deposited in or computed by PubChem | |
Canonical SMILES |
C=CC=P(C1=CC=CC=C1)(C2=CC=CC=C2)C3=CC=CC=C3 | |
Source | PubChem | |
URL | https://pubchem.ncbi.nlm.nih.gov | |
Description | Data deposited in or computed by PubChem | |
Molecular Formula |
C21H19P | |
Source | PubChem | |
URL | https://pubchem.ncbi.nlm.nih.gov | |
Description | Data deposited in or computed by PubChem | |
DSSTOX Substance ID |
DTXSID10333290 | |
Record name | Triphenyl(prop-2-en-1-ylidene)-lambda~5~-phosphane | |
Source | EPA DSSTox | |
URL | https://comptox.epa.gov/dashboard/DTXSID10333290 | |
Description | DSSTox provides a high quality public chemistry resource for supporting improved predictive toxicology. | |
Molecular Weight |
302.3 g/mol | |
Source | PubChem | |
URL | https://pubchem.ncbi.nlm.nih.gov | |
Description | Data deposited in or computed by PubChem | |
CAS No. |
15935-94-1 | |
Record name | Triphenyl(prop-2-en-1-ylidene)-lambda~5~-phosphane | |
Source | EPA DSSTox | |
URL | https://comptox.epa.gov/dashboard/DTXSID10333290 | |
Description | DSSTox provides a high quality public chemistry resource for supporting improved predictive toxicology. | |
Retrosynthesis Analysis
AI-Powered Synthesis Planning: Our tool employs the Template_relevance Pistachio, Template_relevance Bkms_metabolic, Template_relevance Pistachio_ringbreaker, Template_relevance Reaxys, Template_relevance Reaxys_biocatalysis model, leveraging a vast database of chemical reactions to predict feasible synthetic routes.
One-Step Synthesis Focus: Specifically designed for one-step synthesis, it provides concise and direct routes for your target compounds, streamlining the synthesis process.
Accurate Predictions: Utilizing the extensive PISTACHIO, BKMS_METABOLIC, PISTACHIO_RINGBREAKER, REAXYS, REAXYS_BIOCATALYSIS database, our tool offers high-accuracy predictions, reflecting the latest in chemical research and data.
Strategy Settings
Precursor scoring | Relevance Heuristic |
---|---|
Min. plausibility | 0.01 |
Model | Template_relevance |
Template Set | Pistachio/Bkms_metabolic/Pistachio_ringbreaker/Reaxys/Reaxys_biocatalysis |
Top-N result to add to graph | 6 |
Feasible Synthetic Routes
Disclaimer and Information on In-Vitro Research Products
Please be aware that all articles and product information presented on BenchChem are intended solely for informational purposes. The products available for purchase on BenchChem are specifically designed for in-vitro studies, which are conducted outside of living organisms. In-vitro studies, derived from the Latin term "in glass," involve experiments performed in controlled laboratory settings using cells or tissues. It is important to note that these products are not categorized as medicines or drugs, and they have not received approval from the FDA for the prevention, treatment, or cure of any medical condition, ailment, or disease. We must emphasize that any form of bodily introduction of these products into humans or animals is strictly prohibited by law. It is essential to adhere to these guidelines to ensure compliance with legal and ethical standards in research and experimentation.