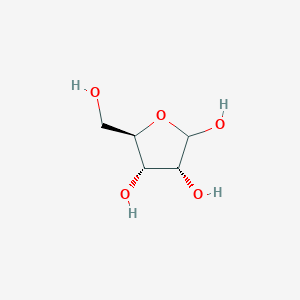
D-ribofuranose
Overview
Description
D-Ribofuranose, a five-membered furanose form of D-ribose, is a critical structural component of RNA, coenzymes (e.g., ATP, NAD+), and synthetic nucleoside analogues. Its β-anomer is universally present in RNA, where the ribose-phosphate backbone facilitates genetic coding and catalysis . The α-anomer, though less common in nature, is synthetically modified to produce derivatives with diverse pharmacological properties, including analgesic, anti-inflammatory, and antiviral activities . The unique stereochemistry of this compound—particularly the cis-diol arrangement at C2 and C3—enables its participation in hydrogen bonding and metal chelation, which are pivotal for biological interactions .
Preparation Methods
Structural and Functional Significance of D-Ribofuranose
This compound exists predominantly in its β-anomeric form, characterized by a five-membered furanose ring with hydroxyl groups at the 2', 3', and 4' positions and a hydroxymethyl group at the 5' position . This configuration is essential for RNA’s structural integrity and its role in genetic transcription. The molecule’s reactivity is influenced by the stereoelectronic effects of its hydroxyl groups, which participate in hydrogen bonding and glycosidic linkage formation . Synthetic access to this compound derivatives, such as 5-deoxy-D-ribofuranose or acetylated analogs, is pivotal for nucleotide analog synthesis, necessitating robust and scalable preparation methods.
Synthesis from D-Ribose via Reductive Deoxygenation
Reductive Displacement of Sulfonate Esters
A seminal method for synthesizing 5-deoxy-D-ribofuranose derivatives involves reductive deoxygenation of sulfonated intermediates. Starting with D-ribose, the 5-hydroxyl group is selectively sulfonated to form methyl 2,3-O-isopropylidene-5-O-sulfonyloxy-β-D-ribofuranoside . Treatment with hydride reagents, such as sodium borohydride or lithium aluminum hydride, induces reductive displacement of the sulfonate group, yielding 5-deoxy-D-ribofuranose intermediates . This step exploits the nucleophilic reactivity of hydrides to cleave the C–O bond, a process facilitated by the electron-withdrawing sulfonate group.
Hydrolysis and Acetylation
Following deoxygenation, the isopropylidene protecting group is removed via acidic hydrolysis, regenerating the free ribofuranose. Subsequent acetylation with acetic anhydride in pyridine affords 1,2,3-tri-O-acetyl-5-deoxy-D-ribofuranose in 56% overall yield from D-ribose . This method’s efficiency stems from its simplicity and compatibility with multi-gram synthesis, making it a preferred route for industrial applications.
Table 1: Reaction Conditions and Yields for Reductive Deoxygenation
Step | Reagents/Conditions | Yield (%) |
---|---|---|
Sulfonation | Methanesulfonyl chloride, pyridine | 85 |
Reductive displacement | NaBH₄, DMF, 80°C | 92 |
Hydrolysis | HCl (aq), reflux | 95 |
Acetylation | Ac₂O, pyridine, rt | 88 |
Acetylation and Protection Strategies for Ribofuranose Derivatives
Acetal Formation and Sequential Acetylation
Industrial-scale synthesis of β-1,2,3,5-tetra-O-acetyl-D-ribofuranose, a key intermediate in antiviral prodrugs, begins with acetal formation. D-Ribose is treated with methanol and sulfuric acid to form methyl β-D-ribofuranoside, which is subsequently acetylated with acetic anhydride . The crude product undergoes acetolysis in acetic acid and sulfuric acid, selectively cleaving the glycosidic bond to yield a mixture of α- and β-anomers of tetra-O-acetyl-D-ribofuranose . Purification via recrystallization from ethyl ether isolates the β-anomer in 57% yield, though scalability is hindered by laborious extraction and evaporation steps .
Challenges in Anomer Separation and Purification
The α- and β-anomers of acetylated ribofuranose exhibit similar physicochemical properties, complicating their separation. Chromatographic methods, while effective, are cost-prohibitive for large-scale production. Recent advances exploit triflic acid-catalyzed equilibration under vacuum distillation, favoring the thermodynamically stable β-anomer . This approach reduces purification steps and improves yields to 75%, demonstrating industrial viability .
Table 2: Optimization of Acetylation and Anomer Separation
Parameter | Conventional Method | Optimized Method |
---|---|---|
Catalyst | H₂SO₄ | Triflic acid |
Temperature (°C) | 25 | 115 |
Yield (β-anomer) | 57% | 75% |
Purification Steps | 8 | 3 |
Mechanistic Insights into Cyclization and Hemiacetal Formation
Acid-Catalyzed Cyclization of Open-Chain D-Ribose
The spontaneous cyclization of open-chain D-ribose to β-D-ribofuranose proceeds via acid-catalyzed hemiacetal formation. Protonation of the aldehyde oxygen enhances electrophilicity, facilitating nucleophilic attack by the 4-hydroxyl group . The resulting oxocarbenium intermediate undergoes ring closure to form a five-membered furanose ring, with the β-anomer predominating due to the anomeric effect .
Methanolysis to Methyl Glycosides
Treatment of β-D-ribofuranose with methanol under acidic conditions yields methyl β-D-ribofuranoside, a stable acetal derivative. This two-step mechanism involves initial hemiacetal protonation, followed by methanol attack at the anomeric carbon . The reaction’s stereoselectivity is governed by steric hindrance and electronic factors, favoring the β-configuration .
Analytical Methods for Monitoring Synthesis
LC-MS Quantification of Intermediates
High-performance liquid chromatography coupled with mass spectrometry (LC-MS) enables precise quantification of D-ribose and 5-deoxy-D-ribofuranose in synthetic mixtures. Using an Acclaim RSL C18 column and acetonitrile-water (35:65) mobile phase, analysts achieve baseline separation with detection limits of 0.0414 μg/mL for D-ribose and 0.0424 μg/mL for 5-deoxy-D-ribofuranose . Calibration curves exhibit excellent linearity (), ensuring method accuracy .
Table 3: Analytical Performance of LC-MS Method
Analyte | Linear Range (μg/mL) | LOD (μg/mL) | RSD (%) |
---|---|---|---|
D-Ribose | 0.08–1.80 | 0.0414 | 1.05 |
5-Deoxy-D-ribofuranose | 0.08–1.84 | 0.0424 | 1.63 |
External Standard Validation
Quantitation via external standard methodology involves preparing calibration solutions at seven concentrations (0.02–0.18%). Precision studies demonstrate interday RSD values below 2%, affirming method robustness .
Comparative Analysis of Synthetic Routes
Yield and Scalability
Reductive deoxygenation offers superior yields (56%) and scalability compared to acetylation-based routes (57–75%) but requires hazardous hydride reagents . Conversely, triflic acid-catalyzed acetylation minimizes purification steps, enhancing industrial feasibility despite higher catalyst costs .
Environmental and Economic Considerations
Hydride-mediated methods generate stoichiometric boron waste, necessitating costly disposal. Acetylation routes, while cleaner, consume large volumes of acetic anhydride and sulfuric acid, contributing to environmental acidification . Lifecycle assessments are critical for selecting sustainable pathways.
Chemical Reactions Analysis
Types of Reactions
D-ribose undergoes several types of chemical reactions, including oxidation, reduction, and substitution reactions.
Common Reagents and Conditions
Oxidation: D-ribose can be oxidized to form ribonic acid using oxidizing agents such as nitric acid or bromine water.
Reduction: Reduction of D-ribose can produce ribitol using reducing agents like sodium borohydride.
Major Products
The major products formed from these reactions include ribonic acid, ribitol, and various glycosides, which have different applications in biochemistry and pharmaceuticals .
Scientific Research Applications
Role in Nucleic Acids
D-ribofuranose is integral to the structure of ribonucleic acids (RNA). Its configuration allows for the formation of the backbone of RNA molecules, which are essential for protein synthesis and genetic information transfer. The presence of hydroxyl groups at specific positions on the ribofuranose ring facilitates interactions with nucleobases, enhancing the stability and functionality of RNA.
Drug Development
2.1 Codrug Design
Recent studies have explored the use of this compound as a core moiety in codrug designs aimed at improving drug delivery systems. For instance, a study demonstrated a codrug incorporating this compound with lenalidomide and paclitaxel. This codrug exhibited enhanced water solubility (685 times that of paclitaxel) and selective release properties due to its phosphodiester moiety, which acts as an enzyme recognition site .
Table 1: Properties of this compound-Based Codrugs
Property | Value |
---|---|
Water Solubility Improvement | 685 times |
Half-life (PTX) | 23.8 hours |
Half-life (LENA) | 26.3 hours |
Overall Yield of Synthesis | 33% |
2.2 Antimicrobial Applications
This compound derivatives have been utilized in developing inhibitors for mycobacterial infections. Specifically, benzothiazinones act as suicide inhibitors of decaprenylphosphoryl-D-ribofuranose 2'-oxidase (DprE1), crucial for mycobacterial cell wall biosynthesis . This highlights the potential of this compound in combating antibiotic-resistant strains.
Biochemical Research
This compound plays a vital role in biochemical research, particularly in the synthesis of modified nucleotides and oligonucleotides. A reliable method for synthesizing 1,2-dideoxy-D-ribofuranose has been developed, allowing for its incorporation into small interfering RNAs (siRNAs). This modification can affect the stability and efficacy of siRNAs in gene silencing applications .
Evolutionary Significance
Studies suggest that N-glycosyl derivatives of β-D-ribofuranose may have contributed to the origins of life on Earth by forming essential components for early biochemical pathways. The evolution from simple ribonucleotides to complex metabolic networks underscores the importance of this compound in biological systems .
Safety and Toxicity Studies
Research has also focused on the safety profile of this compound when administered intravenously. A study evaluated its toxicity in rabbits, indicating that it can be safely used within certain dosage limits . This safety data is crucial for its application in therapeutic settings.
Mechanism of Action
D-ribose plays a crucial role in the synthesis of adenosine triphosphate, which is essential for cellular energy production. It helps ensure there is enough adenosine triphosphate synthesized for the mitochondria to recycle. Unlike other sugars, D-ribose is not normally used by the body for fuel . It also activates adenosine 5’ monophosphate-activated protein kinase, which is involved in cellular energy homeostasis .
Comparison with Similar Compounds
Comparison with Structurally Similar Compounds
D-Ribopyranose vs. D-Ribofuranose
D-Ribopyranose, the six-membered pyranose form of D-ribose, differs in ring size and conformational flexibility. Enzymatic studies show that acetylated β-D-ribofuranose derivatives (e.g., 1,2,3-tri-O-acetyl-5-deoxy-β-D-ribofuranose) undergo regioselective deacetylation at the anomeric position, while ribopyranose derivatives prioritize deacetylation at non-anomeric sites . This distinction impacts their metabolic stability and utility in drug design.
Property | This compound | D-Ribopyranose |
---|---|---|
Ring conformation | Envelope (C2′-endo or C3′-endo) | Chair (⁴C₁) |
Enzymatic hydrolysis | Preferential anomeric deacetylation | Non-anomeric deacetylation |
Biological role | RNA backbone, coenzymes, nucleosides | Rare in nature; synthetic intermediates |
2-Deoxy-D-ribofuranose
2-Deoxy-D-ribofuranose is the sugar backbone of DNA, lacking the hydroxyl group at C2. This modification increases DNA’s resistance to alkaline hydrolysis compared to RNA. Synthetic analogues like 1’-deoxy-1’-(4-fluorophenyl)-β-D-ribofuranose exhibit enhanced stability and unique intermolecular interactions (e.g., F…H hydrogen bonds) in crystal structures, which are absent in hydroxyl-rich this compound .
Arabinofuranose and Xylofuranose
- Arabinofuranose: The C2 epimer of ribofuranose. Mycobacterial cell walls contain arabinofuranose residues in arabinogalactan, making it a target for antitubercular drugs. Inhibitors of decaprenylphosphoryl-β-D-ribofuranose 2'-oxidase (DprE1), such as 3,5-dinitrophenyl triazoles, show cross-reactivity with arabinofuranose biosynthesis enzymes .
- Xylofuranose: The C3 epimer. Unlike ribofuranose, xylofuranose derivatives are rare in nature but serve as precursors for antiviral nucleosides.
Pharmacological Derivatives of this compound
Triazole-Based Derivatives
Triazole-linked this compound derivatives (e.g., compound c from mangrove fungi) exhibit α-glucosidase inhibitory activity (IC₅₀ ~2.5 μM), surpassing acarbose (IC₅₀ ~100 μM) . Modifications at the C3 and C5 positions enhance lipophilicity, improving blood-brain barrier penetration for central analgesic effects .
Acetylated and Benzylated Derivatives
- 1,2,3-Tri-O-acetyl-5-deoxy-β-D-ribofuranose: Synthesized in 56% yield via reductive displacement, this derivative is a key intermediate for antitubercular agents .
- 3-O-Benzyl-4-C-(hydroxymethyl) derivatives : These compounds show 65–72% inhibition in carrageenan-induced paw edema assays, comparable to diclofenac (75%) .
Antiviral Nucleoside Analogues
- Virazole (1-β-D-ribofuranosyl-1,2,4-triazole-3-carboxamide): A broad-spectrum antiviral agent with activity against RNA viruses. Its β-D-ribofuranose moiety is essential for binding viral polymerases .
- 3′-C-Ethynyl-β-D-ribofuranose purine nucleosides: These derivatives inhibit hepatitis C virus (HCV) replication at EC₅₀ values of 0.03–0.1 μM, outperforming ribavirin (EC₅₀ ~10 μM) .
Functional and Structural Comparison Table
Key Research Findings and Discrepancies
- Synthetic Accessibility: Enzymatic synthesis (e.g., porcine liver esterase) achieves regioselective deacetylation in ribofuranose derivatives (yield >70%), whereas chemical methods require harsh conditions .
Biological Activity
D-ribofuranose, a pentose sugar, plays a crucial role in various biological processes, particularly as a component of nucleotides and nucleic acids. Its derivatives have been extensively studied for their potential therapeutic applications due to their diverse biological activities. This article reviews the biological activity of this compound, focusing on its pharmacological properties, toxicity, and implications for therapeutic use.
Overview of this compound
This compound is a naturally occurring sugar that exists predominantly in the furanose form. It is essential for the synthesis of RNA and plays a significant role in cellular metabolism and energy transfer. The biological activity of this compound and its derivatives has been linked to various pharmacological effects, including analgesic, anti-inflammatory, antimicrobial, and cytotoxic properties.
1. Analgesic and Anti-inflammatory Properties
Numerous studies have demonstrated the analgesic and anti-inflammatory activities of this compound derivatives:
- Synthesis of Derivatives : Research has shown that synthesized derivatives of α-D-ribofuranose exhibit significant analgesic effects. For instance, specific analogues were found to reduce pain responses in animal models effectively .
- Molecular Docking Studies : These studies indicated that certain compounds derived from this compound showed strong binding affinities to cyclooxygenase-1 and phospholipase A2, enzymes involved in inflammatory pathways .
Compound | Binding Affinity (kcal/mol) | Activity |
---|---|---|
Compound 3 | -8.1 (COX-1) | Analgesic |
Compound 2 | -9.1 (IL-1R) | Anti-inflammatory |
2. Antimicrobial Activity
This compound derivatives have also been evaluated for their antimicrobial properties:
- Antimicrobial Efficacy : Certain ribofuranosides have demonstrated enhanced antimicrobial activity compared to their parent compounds. This suggests potential applications in developing new antimicrobial agents .
- Mechanism of Action : The antimicrobial effects are thought to stem from the ability of these compounds to disrupt bacterial cell wall synthesis or function .
3. Cytotoxic Effects
The cytotoxicity of this compound derivatives has been explored in various cancer cell lines:
- In Vitro Studies : Some ribofuranosides exhibited significant cytotoxic effects against different cancer cell lines, indicating their potential as anticancer agents .
- Molecular Mechanisms : The mechanisms underlying these effects often involve the induction of apoptosis and inhibition of cellular proliferation pathways .
Toxicity Studies
Despite its therapeutic potential, understanding the toxicity profile of this compound is essential for its clinical application:
- Toxicity Assessment : A study evaluating the intravenous administration of this compound in rabbits found no significant toxic effects at doses up to 420 mg/kg over 28 days. This suggests a favorable safety profile for short-term use .
- Long-term Effects : Further research is needed to assess long-term toxicity and the effects of chronic administration.
Case Studies
Several case studies highlight the clinical applications of this compound:
- Congestive Heart Failure : In clinical settings, D-ribose supplementation has shown promise in improving cardiac function in patients with congestive heart failure by enhancing ATP levels and diastolic performance .
- Fibromyalgia Treatment : Patients with fibromyalgia reported improved symptoms with D-ribose supplementation, showcasing its potential as an adjunct therapy in chronic pain management .
Q & A
Q. Basic: What are the standard synthetic routes for preparing β-D-ribofuranose derivatives, and what analytical methods confirm their purity?
Methodological Answer:
β-D-ribofuranose derivatives are typically synthesized via acetylation, benzoylation, or selective functionalization. For example:
- Acetylation : Reacting D-ribofuranose with acetic anhydride under acidic conditions yields 1,2,3,5-tetra-O-acetyl-β-D-ribofuranose, a common intermediate .
- Bromination : 1-O-acetyl-2,3,5-tri-O-benzoyl-β-D-ribofuranose can undergo bromination using propargyl bromide under phase-transfer conditions (tetrabutylammonium bromide, NaOH) to introduce alkyne groups .
- Acylation : Long-chain fatty acyl derivatives are synthesized using N,N-dicyclohexylcarbodiimide (DCC) as a dehydrating agent .
Analytical Validation :
- Purity : High-performance liquid chromatography (HPLC) and thin-layer chromatography (TLC) are used to monitor reactions.
- Structural Confirmation : Nuclear magnetic resonance (NMR; ¹H, ¹³C) and mass spectrometry (ESI-MS) validate stereochemistry and molecular identity .
Q. Basic: How does the conformational equilibrium of this compound in aqueous solution affect its reactivity in glycosylation reactions?
Methodological Answer:
this compound exists in a dynamic equilibrium of five forms: linear, α/β-furanose, and α/β-pyranose. In water, β-D-ribopyranose dominates (~59%), while β-D-ribofuranose constitutes ~13% . This equilibrium impacts reactivity:
- Glycosylation Efficiency : The furanose form is critical for RNA backbone formation but is less stable in solution. Researchers often stabilize it using protecting groups (e.g., acetyl or benzoyl) to favor furanose ring formation during nucleoside synthesis .
- Detection Methods : NMR (¹H and ¹³C) and circular dichroism (CD) spectroscopy track conformational changes. Computational modeling (e.g., density functional theory) predicts dominant conformers under varying solvent conditions .
Q. Advanced: What strategies resolve contradictions between computational predictions and experimental data regarding the dominant conformers of this compound in non-aqueous solvents?
Methodological Answer:
Discrepancies arise due to solvent polarity, temperature, and hydrogen bonding. To address this:
- Hybrid Experimental-Computational Workflows :
- Experimental : Use low-temperature NMR to "freeze" conformers in non-aqueous solvents (e.g., DMSO-d₆). Compare with X-ray crystallography data for solid-state structures .
- Computational : Apply molecular dynamics (MD) simulations with explicit solvent models to account for solvent-solute interactions. Adjust force fields (e.g., AMBER) to better match experimental NOE (nuclear Overhauser effect) correlations .
- Case Study : In iodination studies, selective functionalization under microwave irradiation altered conformational preferences, requiring recalibration of computational models .
Q. Advanced: How can selective functionalization at the C-2 or C-3 positions of this compound be achieved, and what challenges arise in maintaining stereochemical integrity?
Methodological Answer:
Selective functionalization requires precise control of reaction conditions:
- C-2 Modification : Use bulky protecting groups (e.g., tert-butyldimethylsilyl) at C-3 and C-5 to direct reagents to C-2. For example, iodination with [Ph₃P⁺I]I⁻ under microwave irradiation selectively targets C-2 .
- C-3 Modification : Phase-transfer catalysis (e.g., tetrabutylammonium bromide) with propargyl bromide favors C-5 substitution, leaving C-3 reactive for subsequent acylation .
Challenges :
- Steric Hindrance : Bulky groups can slow reaction kinetics, requiring elevated temperatures or sonication .
- Epimerization Risk : Basic conditions may invert stereochemistry. Use mild acids (e.g., camphorsulfonic acid) for deprotection to retain β-configuration .
Q. Advanced: How do enzymes like DprE1SM and DprE2SM interact with β-D-ribofuranose derivatives, and what mechanistic insights inform inhibitor design?
Methodological Answer:
DprE1SM and DprE2SM are flavin-dependent enzymes involved in epimerizing β-D-ribofuranose derivatives (e.g., decaprenylphosphoryl-β-D-ribofuranose, DPR):
- Mechanism : DprE1SM oxidizes DPR to a 2′-keto intermediate, which DprE2SM reduces using NADH. Substrate analogs like farnesylphosphoryl-β-D-ribofuranose (FPR) mimic DPR and enable kinetic studies via HPLC-MS .
- Inhibitor Design : Suicide inhibitors (e.g., benzothiazinones) covalently bind FAD in DprE1SM. Structure-activity relationship (SAR) studies optimize inhibitors by introducing electron-withdrawing groups to enhance binding affinity .
Properties
IUPAC Name |
(3R,4S,5R)-5-(hydroxymethyl)oxolane-2,3,4-triol | |
---|---|---|
Source | PubChem | |
URL | https://pubchem.ncbi.nlm.nih.gov | |
Description | Data deposited in or computed by PubChem | |
InChI |
InChI=1S/C5H10O5/c6-1-2-3(7)4(8)5(9)10-2/h2-9H,1H2/t2-,3-,4-,5?/m1/s1 | |
Source | PubChem | |
URL | https://pubchem.ncbi.nlm.nih.gov | |
Description | Data deposited in or computed by PubChem | |
InChI Key |
HMFHBZSHGGEWLO-SOOFDHNKSA-N | |
Source | PubChem | |
URL | https://pubchem.ncbi.nlm.nih.gov | |
Description | Data deposited in or computed by PubChem | |
Canonical SMILES |
C(C1C(C(C(O1)O)O)O)O | |
Source | PubChem | |
URL | https://pubchem.ncbi.nlm.nih.gov | |
Description | Data deposited in or computed by PubChem | |
Isomeric SMILES |
C([C@@H]1[C@H]([C@H](C(O1)O)O)O)O | |
Source | PubChem | |
URL | https://pubchem.ncbi.nlm.nih.gov | |
Description | Data deposited in or computed by PubChem | |
Molecular Formula |
C5H10O5 | |
Source | PubChem | |
URL | https://pubchem.ncbi.nlm.nih.gov | |
Description | Data deposited in or computed by PubChem | |
DSSTOX Substance ID |
DTXSID201317333 | |
Record name | D-Ribofuranose | |
Source | EPA DSSTox | |
URL | https://comptox.epa.gov/dashboard/DTXSID201317333 | |
Description | DSSTox provides a high quality public chemistry resource for supporting improved predictive toxicology. | |
Molecular Weight |
150.13 g/mol | |
Source | PubChem | |
URL | https://pubchem.ncbi.nlm.nih.gov | |
Description | Data deposited in or computed by PubChem | |
Physical Description |
Solid | |
Record name | D-Ribose | |
Source | Human Metabolome Database (HMDB) | |
URL | http://www.hmdb.ca/metabolites/HMDB0000283 | |
Description | The Human Metabolome Database (HMDB) is a freely available electronic database containing detailed information about small molecule metabolites found in the human body. | |
Explanation | HMDB is offered to the public as a freely available resource. Use and re-distribution of the data, in whole or in part, for commercial purposes requires explicit permission of the authors and explicit acknowledgment of the source material (HMDB) and the original publication (see the HMDB citing page). We ask that users who download significant portions of the database cite the HMDB paper in any resulting publications. | |
CAS No. |
613-83-2 | |
Record name | D-Ribofuranose | |
Source | CAS Common Chemistry | |
URL | https://commonchemistry.cas.org/detail?cas_rn=613-83-2 | |
Description | CAS Common Chemistry is an open community resource for accessing chemical information. Nearly 500,000 chemical substances from CAS REGISTRY cover areas of community interest, including common and frequently regulated chemicals, and those relevant to high school and undergraduate chemistry classes. This chemical information, curated by our expert scientists, is provided in alignment with our mission as a division of the American Chemical Society. | |
Explanation | The data from CAS Common Chemistry is provided under a CC-BY-NC 4.0 license, unless otherwise stated. | |
Record name | D-Ribofuranose | |
Source | ChemIDplus | |
URL | https://pubchem.ncbi.nlm.nih.gov/substance/?source=chemidplus&sourceid=0000613832 | |
Description | ChemIDplus is a free, web search system that provides access to the structure and nomenclature authority files used for the identification of chemical substances cited in National Library of Medicine (NLM) databases, including the TOXNET system. | |
Record name | D-Ribofuranose | |
Source | EPA DSSTox | |
URL | https://comptox.epa.gov/dashboard/DTXSID201317333 | |
Description | DSSTox provides a high quality public chemistry resource for supporting improved predictive toxicology. | |
Record name | D-Ribose | |
Source | Human Metabolome Database (HMDB) | |
URL | http://www.hmdb.ca/metabolites/HMDB0000283 | |
Description | The Human Metabolome Database (HMDB) is a freely available electronic database containing detailed information about small molecule metabolites found in the human body. | |
Explanation | HMDB is offered to the public as a freely available resource. Use and re-distribution of the data, in whole or in part, for commercial purposes requires explicit permission of the authors and explicit acknowledgment of the source material (HMDB) and the original publication (see the HMDB citing page). We ask that users who download significant portions of the database cite the HMDB paper in any resulting publications. | |
Melting Point |
95 °C | |
Record name | D-Ribose | |
Source | Human Metabolome Database (HMDB) | |
URL | http://www.hmdb.ca/metabolites/HMDB0000283 | |
Description | The Human Metabolome Database (HMDB) is a freely available electronic database containing detailed information about small molecule metabolites found in the human body. | |
Explanation | HMDB is offered to the public as a freely available resource. Use and re-distribution of the data, in whole or in part, for commercial purposes requires explicit permission of the authors and explicit acknowledgment of the source material (HMDB) and the original publication (see the HMDB citing page). We ask that users who download significant portions of the database cite the HMDB paper in any resulting publications. | |
Retrosynthesis Analysis
AI-Powered Synthesis Planning: Our tool employs the Template_relevance Pistachio, Template_relevance Bkms_metabolic, Template_relevance Pistachio_ringbreaker, Template_relevance Reaxys, Template_relevance Reaxys_biocatalysis model, leveraging a vast database of chemical reactions to predict feasible synthetic routes.
One-Step Synthesis Focus: Specifically designed for one-step synthesis, it provides concise and direct routes for your target compounds, streamlining the synthesis process.
Accurate Predictions: Utilizing the extensive PISTACHIO, BKMS_METABOLIC, PISTACHIO_RINGBREAKER, REAXYS, REAXYS_BIOCATALYSIS database, our tool offers high-accuracy predictions, reflecting the latest in chemical research and data.
Strategy Settings
Precursor scoring | Relevance Heuristic |
---|---|
Min. plausibility | 0.01 |
Model | Template_relevance |
Template Set | Pistachio/Bkms_metabolic/Pistachio_ringbreaker/Reaxys/Reaxys_biocatalysis |
Top-N result to add to graph | 6 |
Feasible Synthetic Routes
Disclaimer and Information on In-Vitro Research Products
Please be aware that all articles and product information presented on BenchChem are intended solely for informational purposes. The products available for purchase on BenchChem are specifically designed for in-vitro studies, which are conducted outside of living organisms. In-vitro studies, derived from the Latin term "in glass," involve experiments performed in controlled laboratory settings using cells or tissues. It is important to note that these products are not categorized as medicines or drugs, and they have not received approval from the FDA for the prevention, treatment, or cure of any medical condition, ailment, or disease. We must emphasize that any form of bodily introduction of these products into humans or animals is strictly prohibited by law. It is essential to adhere to these guidelines to ensure compliance with legal and ethical standards in research and experimentation.