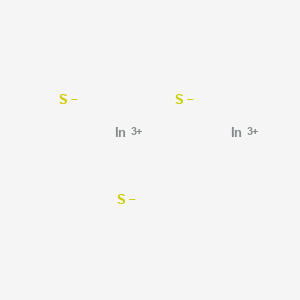
Indium sulfide (In2S3)
- Click on QUICK INQUIRY to receive a quote from our team of experts.
- With the quality product at a COMPETITIVE price, you can focus more on your research.
Overview
Description
Indium sulfide (In₂S₃) is a III–VI group semiconductor with a tunable bandgap ranging from 2.0 to 2.3 eV, depending on its crystalline phase (α, β, or γ) and synthesis method . It is characterized by low toxicity, high absorption coefficients (>10⁴ cm⁻¹), and excellent photoconductive properties . These attributes make it suitable for diverse applications, including:
- Solar cells: As a non-toxic buffer layer alternative to CdS in CIGS-based photovoltaics .
- Supercapacitors: High specific capacitance (258 F/g) when hybridized with carbon nanotubes .
- Photocatalysis: Efficient degradation of pollutants under visible light due to its narrow bandgap .
- Optoelectronics: Use in photodetectors and flexible devices .
In₂S₃ can be synthesized via thermal evaporation, chemical bath deposition (CBD), spray pyrolysis, and solvothermal methods, yielding thin films, nanoflakes, or quantum dots (QDs) .
Preparation Methods
Hydrothermal Synthesis
Hydrothermal synthesis remains a cornerstone for producing In₂S₃ microspheres with controlled morphologies. A template-free and surfactant-less approach at 150°C using indium nitrate (In(NO₃)₃) and thioacetamide (TAA) yielded β-In₂S₃ microspheres composed of nanoflakes or nanobricks, depending on the indium precursor . X-ray diffraction (XRD) confirmed the tetragonal phase purity, while X-ray photoelectron spectroscopy (XPS) validated the stoichiometric In:S ratio of 2:3.
Role of Precursors
Indium chloride (InCl₃) produced nanoflakes, whereas In(NO₃)₃ led to nanobricks due to differences in precursor hydrolysis rates and sulfur release kinetics . The slower release of S²⁻ from TAA in nitrate systems facilitated oriented attachment into brick-like structures, whereas chloride systems promoted flake formation.
Photocatalytic Performance
The microspheres degraded 95% of methylene blue (MB) and 89% of crystal violet (CV) under visible light in 120 minutes, attributed to hydroxyl radical (- OH) generation confirmed via terephthalic acid photoluminescence assays . Reusability tests showed no morphological degradation after five cycles, underscoring structural stability.
Microwave-Assisted Synthesis
Microwave irradiation enables rapid, energy-efficient synthesis of hierarchical In₂S₃ structures. A 10-minute reaction at 90°C with citric acid (CA) as a pH regulator produced 500–600 nm thornball-like β-In₂S₃ . CA coordinated with In³⁺ to form intermediate complexes, guiding the self-assembly of nanoflakes into spherical superstructures.
Size-Dependent Properties
Varying irradiation times (5–15 minutes) modulated particle size:
-
5 minutes : 400–500 nm spheres with thin nanoflakes (Fig. 2a) .
-
10 minutes : Optimal 500–600 nm spheres exhibiting maximal hydrophilicity and surface negative charges .
-
15 minutes : Aggregated microspheres with reduced surface area.
The 10-minute sample (In₂S₃-10) achieved complete rhodamine B (RhB) degradation in 25 minutes, outperforming literature precedents . Fourier-transform infrared (FT-IR) spectroscopy linked this to residual CA enhancing dye adsorption via carboxylate interactions.
Ultrasonic Dispersion
Ultrasonic methods offer a rapid, surfactant-free route to In₂S₃ nanoparticles. Sonication of bulk indium and sulfur in ethanol produced 10–20 nm nanoparticles within minutes . Transmission electron microscopy (TEM) revealed spherical morphologies, while XRD confirmed cubic phase formation.
Mechanistic Insights
Ultrasound-induced cavitation generated localized hotspots (∼5000 K), accelerating sulfur reduction and In₂S₃ nucleation . Thermogravimetric analysis (TGA) showed a 2.5% weight loss up to 600°C, indicating high thermal stability.
Tribological Applications
As a lubricant additive, In₂S₃ nanoparticles reduced friction coefficients by 40% compared to base oils, attributed to their lamellar structure facilitating interlayer sliding .
Solvothermal Core-Shell Fabrication
In₂S₃@MIL-125(Ti) core-shell microparticles were synthesized via a one-pot solvothermal method at 150°C . MIL-125(Ti), a titanium-based metal-organic framework (MOF), served as a scaffold for In₂S₃ growth, enhancing charge separation and adsorption capacity.
Structural Characterization
High-resolution TEM (HRTEM) revealed a lattice spacing of 0.189 nm, corresponding to the (440) plane of cubic In₂S₃ (Fig. 2h) . Energy-dispersive X-ray spectroscopy (EDS) confirmed a 2:3 In:S ratio, while XPS detected Ti³⁺ species, indicating electron transfer from In₂S₃ to MIL-125(Ti).
Enhanced Photocatalysis
The core-shell structure degraded 98% of tetracycline in 90 minutes under visible light, outperforming pure In₂S₃ (72%) . Transient photocurrent measurements showed a 3.2-fold increase in charge carrier density, linked to the MOF’s electron-trapping ability.
Single-Source Precursor Thermolysis
Thermolysis of a heteroleptic indium complex, [In(NMe₂CH(Me)CH₂S)₃], in oleylamine at 150°C produced ultrathin In₂S₃ nanosheets (NSs) with 1.76 nm thickness . Atomic force microscopy (AFM) confirmed quantum confinement effects, with a blue-shifted bandgap of 2.8 eV compared to bulk In₂S₃ (2.0 eV).
Optoelectronic Properties
Photoluminescence (PL) spectra exhibited a strong emission peak at 460 nm, suggesting applications in blue-light-emitting diodes . Prototype photoelectrochemical cells showed a photocurrent density of 1.2 mA/cm² under AM 1.5G illumination, rivaling commercial TiO₂-based cells.
Comparative Analysis of Synthesis Methods
Chemical Reactions Analysis
Direct Elemental Combination
-
Reaction : 2In + 3S → In₂S₃
Chemical Vapor Deposition (CVD)
-
Reaction : In (film) + S (vapor) → β-In₂S₃
Decomposition and Phase Transitions
In₂S₃ undergoes temperature-dependent structural transformations:
Acid Reactions
-
Mineral Acids :
-
Nitric Acid :
Base Reactions
-
Sulfide Solutions :
CO₂ Electroreduction
-
Catalyst : Zn-doped In₂S₃ (ZnIn₂S₄)
-
Reaction : CO₂ + H₂O → HCOO⁻ (formate)
-
Performance :
-
-
Mechanism : Zn enhances In–S bond covalency, stabilizing sulfur sites for H₂O activation .
Hydrogen Production
Scientific Research Applications
Indium sulfide has a wide range of scientific research applications due to its unique properties:
Optoelectronics: It is used in the fabrication of photodetectors and solar cells due to its suitable band gap and photoconductive properties.
Photocatalysis: Indium sulfide-based photocatalysts are employed in hydrogen production and water cleaning applications.
Biological Imaging: Indium sulfide quantum dots are used in biological imaging due to their excellent optical properties.
Gas Sensors: Indium sulfide is used in gas sensors for detecting various gases.
Mechanism of Action
The mechanism by which indium sulfide exerts its effects is primarily through its semiconductor properties. In optoelectronic applications, indium sulfide absorbs light and generates electron-hole pairs, which are then separated and collected to produce an electric current . In photocatalytic applications, indium sulfide absorbs visible light, generating electron-hole pairs that participate in redox reactions to produce hydrogen gas or degrade pollutants .
Comparison with Similar Compounds
Cadmium Sulfide (CdS)
CdS has been widely used as a buffer layer in thin-film solar cells but faces criticism due to its high toxicity and environmental hazards.
In₂S₃ outperforms CdS in environmental safety and demonstrates comparable optoelectronic performance. For instance, In₂S₃-based solar cells achieve 18.22% power conversion efficiency (PCE) as electron transport materials (ETMs), rivaling traditional CdS-based devices .
Zinc Sulfide (ZnS)
Property | In₂S₃ | ZnS |
---|---|---|
Bandgap (eV) | 2.0–2.3 | ~3.5 |
Photocatalytic Activity | High under visible light | Limited to UV light |
Synthesis | Solvothermal, SILAR | Hydrothermal, CBD |
In₂S₃’s narrower bandgap enables superior visible-light utilization. For example, In₂S₃/MoS₂ heterostructures degrade 95% of methyl orange under visible light, outperforming ZnS-based catalysts .
Molybdenum Disulfide (MoS₂)
MoS₂, a transition metal dichalcogenide (TMDC), shares layered structures with γ-In₂S₃ but differs in electronic properties.
Property | In₂S₃ | MoS₂ |
---|---|---|
Bandgap (eV) | 2.0–2.3 | 1.2–1.8 (layer-dependent) |
Conductivity | High (layered structure) | Moderate |
Supercapacitance | 258 F/g (In₂S₃-SWCNT composite) | ~170 F/g (MoS₂-rGO composite) |
In₂S₃’s higher conductivity and redox activity make it preferable for energy storage. Its composite with SWCNTs retains 96.8% capacitance after 3,000 cycles, showcasing robust stability .
Other Transition Metal Sulfides (TMSs)
TMSs like CuS and SnS₂ are explored for energy storage and catalysis.
Compound | Bandgap (eV) | Key Application | Performance vs. In₂S₃ |
---|---|---|---|
CuS | 1.2–2.2 | Supercapacitors | Lower cyclic stability |
SnS₂ | 2.2–2.4 | Photodetectors | Comparable bandgap, less studied |
In₂S₃’s layered structure and defect-tolerant properties enhance its versatility across applications .
Challenges and Future Outlook
- Bandgap Controversy: Discrepancies exist between reports of direct (2.3 eV) and indirect (2.0 eV) bandgaps, likely due to phase variations or quantum confinement in nanostructures .
- Environmental Hazards : While less toxic than CdS, In₂S₃ requires careful handling due to risks of skin/eye irritation and sulfur oxide release under decomposition .
- Scalability : High-temperature synthesis (e.g., 550°C for thin films) limits cost-effectiveness .
Tables
Table 1: Bandgap and Application Comparison
Compound | Bandgap (eV) | Toxicity | Key Applications |
---|---|---|---|
In₂S₃ | 2.0–2.3 | Low | Solar cells, supercapacitors |
CdS | ~2.4 | High | Solar cells (buffer layer) |
ZnS | ~3.5 | Moderate | UV photocatalysis |
MoS₂ | 1.2–1.8 | Low | Electronics, catalysis |
Table 2: Supercapacitor Performance
Material | Specific Capacitance (F/g) | Cycle Stability |
---|---|---|
In₂S₃-SWCNT composite | 258 at 1 A/g | 96.8% (3k cycles) |
MoS₂-rGO composite | ~170 | 90% (5k cycles) |
Biological Activity
Indium sulfide (In₂S₃) is an inorganic compound that has garnered attention in various fields, including materials science and biomedicine, due to its unique properties and biological activities. This article explores the biological activity of In₂S₃, focusing on its toxicity, potential therapeutic applications, and interactions with biological systems.
Properties and Structure
Indium sulfide exists in several polymorphic forms, with β-In₂S₃ being the most stable at room temperature. It has a defect spinel structure that contributes to its electronic properties, making it a candidate for various applications including photocatalysis and optoelectronics . The compound is characterized by its low toxicity compared to other metal sulfides, such as cadmium sulfide, which is crucial for its biological applications .
Toxicity Assessment
Research indicates that In₂S₃ exhibits low toxicity levels when compared to other semiconductor materials. In vitro studies have shown minimal adverse effects on cell viability, making it a safer alternative for biomedical applications . The compound's interactions with biological systems are influenced by its size and surface properties. For instance, nanoscale In₂S₃ particles have displayed different biological behaviors compared to bulk materials due to their increased surface area and reactivity.
Property | Value |
---|---|
Toxicity Level | Low |
Average Particle Size | ~3.6 nm (nanoparticles) |
Stability | High (especially β-In₂S₃) |
Photocatalytic Activity
In₂S₃ is recognized for its photocatalytic properties, particularly in environmental remediation. Studies have demonstrated that In₂S₃ can effectively degrade organic pollutants under visible light irradiation. This photocatalytic activity is attributed to its ability to generate reactive oxygen species (ROS), which can interact with various biological molecules . The degradation efficiency of In₂S₃ can be significantly enhanced when combined with other materials like MoS₂, leading to improved charge carrier dynamics and pollutant degradation rates .
Case Studies
- Chirality Induction in Nanoparticles : A recent study reported the synthesis of chiral In₂S₃ nanoparticles using cysteine as a chiral agent. These nanoparticles exhibited significant circular dichroism signals, indicating successful chirality transfer from the ligand to the In₂S₃ matrix. This finding opens new avenues for using chiral nanomaterials in drug delivery and biosensing applications .
- Photocatalytic Degradation of Pollutants : Another investigation focused on the photocatalytic capabilities of In₂S₃ nanostructures in degrading methylene blue (MB) and oxytetracycline hydrochloride (OTC-HCl). The study found that optimized In₂S₃-MoS₂ nanohybrids could decompose over 97% of MB within 8 minutes under sunlight exposure, highlighting their potential for environmental cleanup .
Research Findings
- Low Toxicity : Compared to cadmium and lead compounds, In₂S₃ poses a significantly lower risk of secondary pollution during photoconversion processes .
- Enhanced Photocatalytic Efficiency : Modifications such as doping with cobalt or creating heterostructures with other materials have been shown to improve the photocatalytic efficiency of In₂S₃ significantly .
- Applications in Medicine : Due to its low toxicity and ability to generate ROS, In₂S₃ is being explored for applications in photothermal therapy and as a contrast agent in imaging techniques .
Q & A
Q. Basic: What are the standard synthesis methods for high-purity In2S3, and how do experimental parameters influence phase purity?
Methodological Answer:
In2S3 is commonly synthesized via solid-state reactions. A validated protocol involves heating stoichiometric ratios of indium (≥99.999% purity) and sulfur (≥99.999% purity) in evacuated quartz ampoules at 650–850°C for 24–72 hours . Excess sulfur (4–5 atomic%) is added to compensate for sublimation losses and ensure phase purity. Post-synthesis, controlled cooling rates (1–5°C/min) prevent secondary phase formation. Characterization via X-ray diffraction (XRD) and Raman spectroscopy is critical to confirm α-, β-, or γ-phase dominance, which depends on annealing temperature and sulfur partial pressure.
Key Parameters Table:
Parameter | Typical Range | Impact on Phase |
---|---|---|
Temperature | 650–850°C | Higher temps favor β-phase |
Sulfur Excess | 4–5% | Prevents In-rich phases |
Cooling Rate | 1–5°C/min | Affects crystallinity |
Q. Basic: What structural and electronic properties make In2S3 suitable for optoelectronic applications?
Methodological Answer:
In2S3 exhibits a tunable bandgap (2.0–2.3 eV for β-phase) and high electron mobility (~10 cm²/V·s), making it ideal for thin-film solar cells and photodetectors. Its defect-tolerant structure allows n-type conductivity via sulfur vacancies, which can be modulated through doping (e.g., Sn or Al). UV-Vis spectroscopy and Hall effect measurements are essential to correlate synthesis conditions with optoelectronic performance .
Q. Advanced: How can researchers resolve contradictions in reported bandgap values of In2S3 across studies?
Methodological Answer:
Discrepancies arise from variations in synthesis methods (e.g., sulfurization time) and characterization techniques. For example, UV-Vis absorption often overestimates bandgaps due to excitonic effects, while photoluminescence (PL) spectroscopy provides more accurate values. To address contradictions:
Standardize Synthesis: Replicate conditions from conflicting studies (e.g., temperature gradients, precursor ratios).
Cross-Validate Techniques: Combine UV-Vis, PL, and ellipsometry to minimize measurement artifacts.
Statistical Analysis: Use meta-analysis to identify outliers and correlate bandgap trends with synthesis parameters .
Example Data Comparison:
Study | Bandgap (eV) | Method | Synthesis Temp. |
---|---|---|---|
A | 2.1 | UV-Vis | 700°C |
B | 2.3 | PL | 750°C |
Q. Advanced: What strategies optimize In2S3 as a buffer layer in CIGS solar cells to replace toxic CdS?
Methodological Answer:
In2S3 outperforms CdS in eco-friendliness but requires interface engineering for comparable efficiency (~16% vs. 20% for CdS). Key strategies:
- Thickness Optimization: 50–100 nm layers balance light transmission and carrier collection.
- Doping: Incorporate oxygen or fluorine to reduce interface recombination.
- Post-Deposition Annealing: 300–400°C in sulfur atmosphere improves crystallinity.
SCAPS-1D simulations can model band alignment and predict performance under varying parameters .
Performance Metrics Table:
Buffer Layer | Efficiency (%) | Toxicity | Stability (85°C/85% RH) |
---|---|---|---|
CdS | 20 | High | Moderate |
In2S3 | 16 | None | High |
Q. Advanced: How to design experiments investigating In2S3’s stability under environmental stress for photovoltaic applications?
Methodological Answer:
Adopt a tiered experimental design:
Accelerated Aging: Expose samples to 85°C/85% relative humidity (RH) for 1,000 hours.
In Situ Characterization: Use XRD and SEM pre/post aging to detect phase changes or delamination.
Electrochemical Impedance Spectroscopy (EIS): Quantify degradation in charge transport properties.
Control Variables: Compare encapsulated vs. unencapsulated layers to isolate degradation pathways. Cross-reference findings with long-term field studies to validate lab-scale results .
Q. Basic: What safety protocols are critical when handling In2S3 in laboratory settings?
Methodological Answer:
In2S3 poses inhalation risks (Xn hazard classification). Mandatory protocols include:
Properties
CAS No. |
12030-14-7 |
---|---|
Molecular Formula |
InS |
Molecular Weight |
146.89 g/mol |
IUPAC Name |
sulfanylideneindium |
InChI |
InChI=1S/In.S |
InChI Key |
GKCNVZWZCYIBPR-UHFFFAOYSA-N |
SMILES |
[S-2].[S-2].[S-2].[In+3].[In+3] |
Canonical SMILES |
S=[In] |
Key on ui other cas no. |
12030-24-9 37231-03-1 |
Pictograms |
Irritant |
Origin of Product |
United States |
Disclaimer and Information on In-Vitro Research Products
Please be aware that all articles and product information presented on BenchChem are intended solely for informational purposes. The products available for purchase on BenchChem are specifically designed for in-vitro studies, which are conducted outside of living organisms. In-vitro studies, derived from the Latin term "in glass," involve experiments performed in controlled laboratory settings using cells or tissues. It is important to note that these products are not categorized as medicines or drugs, and they have not received approval from the FDA for the prevention, treatment, or cure of any medical condition, ailment, or disease. We must emphasize that any form of bodily introduction of these products into humans or animals is strictly prohibited by law. It is essential to adhere to these guidelines to ensure compliance with legal and ethical standards in research and experimentation.