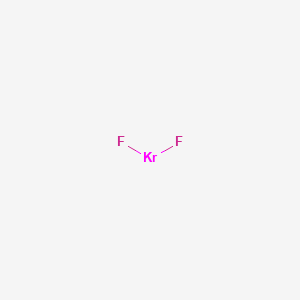
Krypton difluoride
- Click on QUICK INQUIRY to receive a quote from our team of experts.
- With the quality product at a COMPETITIVE price, you can focus more on your research.
Overview
Description
Krypton difluoride (KrF₂), the first discovered binary compound of krypton, was synthesized in 1963 via ultraviolet photolysis of fluorine in a solid argon-krypton matrix at 20 K . Its preparation requires extreme conditions, such as low temperatures (−196°C) and electric discharge or irradiation of krypton-fluorine mixtures, due to its thermodynamic instability . KrF₂ is a colorless, volatile solid that decomposes above −80°C, with a linear molecular structure (Kr–F bond length: 188.9 pm) featuring three-center, four-electron (3c-4e) bonding . Despite its low atomization energy (98 kJ·mol⁻¹), which is weaker than elemental fluorine’s dissociation energy (153 kJ·mol⁻¹), KrF₂ is a potent fluorinating agent and oxidizing agent, surpassing even fluorine in reactivity .
Scientific Research Applications
Chemical Synthesis and Oxidizing Agent
Krypton difluoride is recognized as one of the strongest oxidizers available, comparable to platinum hexafluoride and dioxygen difluoride. This property allows it to oxidize various substances effectively, including the oxidation of plutonium(IV) to plutonium hexafluoride. The compound has been utilized in the preparation of several high-valent inorganic compounds, such as:
- Silver trifluoride (AgF₃)
- Osmium tetrafluoride (cis-OsO₂F₄)
- Other halogenated compounds (C₁F₃ and BrF₃)
These reactions not only demonstrate the versatility of this compound in synthetic chemistry but also highlight its potential in producing novel materials with unique properties .
Coordination Chemistry
Recent studies have shown that this compound can act as a ligand in coordination compounds. For example, a coordination compound involving this compound and a bromine atom has been synthesized. This compound was characterized using X-ray diffraction, revealing a trigonal pyramidal geometry that indicates more covalent character than previously observed in other krypton compounds. Such findings suggest that this compound may play a role in expanding the understanding of noble gas chemistry and coordination complexes .
Laser Technology
This compound has applications in laser technology, particularly as a precursor for chemically pumped KrF lasers. These lasers are used in various applications, including lithography for semiconductor manufacturing and medical procedures. The ability of this compound to be stored as a solid precursor enhances its practicality for use in these high-tech environments .
Environmental and Safety Applications
This compound is being explored for its potential use in decontaminating equipment used in plutonium processing. Its strong oxidizing properties can facilitate the breakdown of hazardous materials, making it valuable for environmental safety applications .
Research on Novel Compounds
The synthesis of this compound has opened avenues for research into new compounds that incorporate noble gases. The unique properties of this compound allow chemists to explore its interactions with other elements, potentially leading to the discovery of new materials with desirable characteristics for various industrial applications .
Summary Table of Applications
Application Area | Description |
---|---|
Chemical Synthesis | Strong oxidizer for producing high-valent inorganic compounds |
Coordination Chemistry | Acts as a ligand in coordination complexes |
Laser Technology | Precursor for chemically pumped KrF lasers |
Environmental Safety | Used for decontaminating plutonium processing equipment |
Research on Novel Compounds | Facilitates exploration of new materials incorporating noble gases |
Case Studies
- Synthesis of Silver Trifluoride : A study demonstrated the use of this compound to synthesize silver trifluoride efficiently, showcasing its role as an oxidizing agent in fluorine chemistry.
- Coordination Compound Characterization : Researchers synthesized a novel compound involving this compound acting as a ligand to bromine, revealing insights into its bonding characteristics and potential applications in materials science.
- Laser Development : Investigations into the use of this compound as a precursor for laser technologies have led to advancements in high-resolution lithography techniques essential for semiconductor fabrication.
Chemical Reactions Analysis
Oxidation of Metals
KrF₂ acts as a potent oxidizer, surpassing elemental fluorine in redox potential (+3.5 V for KrF₂/Kr) .
Reaction | Conditions | Products | References |
---|---|---|---|
7KrF2+2Au→2KrF+AuF6−+5Kr | Room temperature | Au(V) hexafluoroaurate(V) | |
KrF2+Ag→AgF3 | Not specified | Silver(III) fluoride |
-
Gold Oxidation : KrF₂ oxidizes gold to its +5 oxidation state, forming KrF+AuF6−, which decomposes at 60°C to release AuF5, Kr, and F2 .
-
Silver Oxidation : Metallic silver reacts to form AgF3, a rare +3 oxidation state compound .
Reactions with Nonmetals
KrF₂ fluorinates and oxidizes nonmetals, including xenon and halogens:
Reaction | Conditions | Products | References |
---|---|---|---|
3KrF2+Xe→XeF6+3Kr | Room temperature | Xenon hexafluoride | |
KrF2+SbF5→KrF+SbF6− | Low temperatures | KrF+ salt | |
KrF+SbF6−+BrF5→BrF6++Kr+SbF5 | Not specified | BrF6+ cation |
-
Xenon Fluoridation : KrF₂ converts xenon to XeF6, the highest fluoride of xenon .
-
Cation Generation : Reactions with strong Lewis acids (e.g., SbF5) yield KrF+, which further oxidizes BrF5 and ClF5 to BrF6+ and ClF6+ .
Thermal and Radiochemical Decomposition
KrF₂ is thermally unstable and decomposes under various conditions:
Process | Conditions | Products | References |
---|---|---|---|
KrF2→Kr+F2 | > -80°C | Krypton and fluorine gas | |
KrF2γ raysKrF∙ | 77 K | Krypton monofluoride radical |
-
Thermal Decomposition : At room temperature, KrF₂ decomposes at 10% per hour .
-
Radical Formation : γ-irradiation at 77 K produces the violet KrF∙ radical, stable only below 120 K .
Bonding and Energetics
The weak Kr–F bond underpins KrF₂’s reactivity:
Property | Value | Reference |
---|---|---|
Bond dissociation energy (Kr–F) | 11 kcal/mol | |
Ionization energy (KrF2) | 13.06–13.71 eV | |
Heat of formation (ΔHf∘) | +14.4 ± 0.8 kcal/mol (gas phase) |
High-Pressure Reactivity
Under extreme pressures (>50 GPa), computational studies predict novel fluorides like KrF4 and KrF with direct Kr–Kr bonds .
Q & A
Q. Basic: What are the primary synthesis methods for krypton difluoride, and how do they differ in experimental setup?
This compound is synthesized under cryogenic conditions due to its thermodynamic instability. The most common methods include:
- UV Photolysis : Irradiating a mixture of krypton and fluorine gas in liquid fluorine at temperatures below −196°C. This method is optimized for scalability, yielding up to 6 g/hr .
- Electric Discharge : Applying high-voltage discharges to a krypton-fluorine mixture at low temperatures, which generates F· radicals for reaction .
- Hot-Wire Thermolysis : Heating a tungsten filament in a fluorine-rich environment to produce F· radicals, enabling controlled synthesis .
Methodological considerations include temperature control, fluorine gas purity, and radiation intensity to minimize decomposition.
Q. Advanced: How can researchers optimize KrF₂ synthesis yields while mitigating thermodynamic instability?
Optimization requires balancing reaction kinetics and thermodynamic constraints:
- Liquid Fluorine Solvent : UV photolysis in liquid fluorine enhances radical density, improving reaction rates and yields (1.22–6 g/hr) .
- Radical Trapping : Introducing inert matrices (e.g., argon) stabilizes reactive intermediates, reducing side reactions .
- In Situ Monitoring : Infrared spectroscopy tracks KrF₂ formation and decomposition, allowing real-time adjustments to reaction parameters .
Advanced studies should also explore pulsed laser photolysis or catalytic F· generation to enhance efficiency.
Q. Basic: What spectroscopic and crystallographic techniques are critical for characterizing KrF₂?
- Vibrational Spectroscopy : High-resolution IR and Raman spectroscopy identify Kr–F stretching modes (e.g., symmetric stretch at ~449 cm⁻¹), confirming linear geometry .
- 19F NMR : Detects fluorine environments, though limited by KrF₂’s low stability at ambient temperatures .
- Gas-Phase Electron Diffraction : Measures bond length (1.882–1.889 Å) and validates centrosymmetric structure .
- X-ray Crystallography : Rarely applied due to KrF₂’s volatility, but single-crystal studies confirm solid-state packing .
Q. Advanced: How do discrepancies arise between experimental and computational models of KrF₂’s vibrational spectra?
Discrepancies stem from approximations in density functional theory (DFT) calculations. For example:
- Anharmonicity : Experimental spectra include anharmonic effects, while standard DFT models assume harmonic oscillations, leading to deviations in peak positions .
- Isotopic Effects : Natural krypton isotopic mixtures (e.g., ⁸⁴Kr, ⁸⁶Kr) complicate spectral assignments, requiring isotopic enrichment for precise comparisons .
Advanced studies should incorporate relativistic corrections and coupled-cluster theory to improve agreement with experimental data.
Q. Basic: What safety protocols are essential for handling KrF₂ in laboratory settings?
- Cryogenic Storage : KrF₂ must be stored below −78°C to prevent exothermic decomposition into Kr and F₂ .
- Inert Atmospheres : Use gloveboxes with argon or nitrogen to avoid moisture-induced hydrolysis .
- Personal Protective Equipment (PPE) : Fluorine-resistant materials and face shields are mandatory due to F₂ gas toxicity .
Q. Advanced: How can researchers reconcile conflicting reports on higher krypton fluorides (e.g., KrF₄)?
Early studies misidentified KrF₂ as KrF₄ due to inadequate spectroscopic resolution . Contemporary analyses suggest:
- Synthetic Conditions : Higher fluorides may form transiently under extreme pressures or plasma conditions but lack thermodynamic stability .
- Matrix Isolation : Trapping metastable species in inert matrices (e.g., solid argon) could enable characterization via low-temperature IR spectroscopy .
Advanced validation requires high-pressure synthesis coupled with synchrotron X-ray diffraction.
Q. Basic: What role does KrF₂ play as a fluorinating and oxidizing agent in inorganic synthesis?
KrF₂ oxidizes and fluorinates substrates via two pathways:
- Direct Fluorination : Transfers F⁻ to electrophilic centers (e.g., converting Au to AuF₅⁻) .
- Radical Mechanisms : Generates F· radicals under UV exposure, enabling C–F bond formation in organic compounds .
Its strong oxidative power (E° ~3.5 V) makes it useful in synthesizing high-valent metal fluorides .
Q. Advanced: What computational approaches predict KrF₂’s reactivity with novel substrates?
- Molecular Orbital Theory : Analyses of KrF₂’s LUMO (σ* Kr–F) identify electrophilic attack sites .
- Reactivity Indices : Fukui function calculations predict nucleophilic regions in target molecules susceptible to fluorination .
- Kinetic Modeling : Transition-state simulations assess activation barriers for fluorination pathways, guiding experimental design .
Q. Basic: How does KrF₂’s thermodynamic instability influence experimental design?
- Short Reaction Times : Rapid quenching (e.g., liquid nitrogen cooling) prevents decomposition post-synthesis .
- Low-Temperature Techniques : Reactions are conducted in cryostats or cold traps to maintain stability .
Q. Advanced: What strategies stabilize KrF₂ derivatives for extended studies?
- Coordination Complexes : Binding KrF₂ to Lewis acids (e.g., SbF₅) forms stable salts like [KrF⁺][SbF₆⁻] .
- Encapsulation : Host-guest systems (e.g., metal-organic frameworks) isolate KrF₂ from reactive environments .
- Isotopic Substitution : Using ⁸⁶Kr reduces vibrational entropy, marginally enhancing stability .
Comparison with Similar Compounds
Xenon Difluoride (XeF₂)
- Synthesis & Stability: XeF₂ forms under milder conditions (e.g., Xe and F₂ heated to 400°C) and is stable at room temperature, unlike KrF₂ . This stability arises from xenon’s larger atomic size and stronger relativistic effects, which stabilize higher oxidation states.
- Structure & Bonding: XeF₂ also adopts a linear geometry (Xe–F bond: 197 pm) with 3c-4e bonding, but its Xe–F bond energy (133 kJ·mol⁻¹) is higher than Kr–F (98 kJ·mol⁻¹), contradicting its greater thermodynamic stability . This anomaly is attributed to xenon’s superior electron shielding and lower ionization energy.
- Reactivity : While XeF₂ is a strong fluorinating agent, it is less reactive than KrF₂. XeF₂ is widely used in organic synthesis and semiconductor etching, whereas KrF₂ is primarily a laboratory curiosity .
Xenon Tetrafluoride (XeF₄) and Hexafluoride (XeF₆)
- Oxidation States: Xenon exhibits diverse oxidation states (+2, +4, +6), forming XeF₄ (square planar) and XeF₆ (distorted octahedral).
Radon Difluoride (RnF₂)
- Synthesis Challenges : RnF₂ is theorized but rarely studied due to radon’s radioactivity (half-life: 3.8 days for ²²²Rn). It is postulated to form under similar conditions as KrF₂ but with even greater instability .
Data Tables
Table 1: Structural and Thermodynamic Properties
Key Research Findings
All known krypton compounds derive from KrF₂ .
Paradoxical Bond Strength : Despite Kr–F bonds (133 kJ·mol⁻¹) being stronger than Xe–F (50 kJ·mol⁻¹), KrF₂’s overall instability stems from weaker lattice energies and higher entropy of decomposition .
Hypervalent Bonding : KrF₂’s 3c-4e bonding involves hypervalent krypton, a concept debated since Langmuir and Lewis proposed conflicting models (octet vs. expanded octet) .
Preparation Methods
Electrical Discharge Synthesis
Methodology and Reaction Conditions
The electrical discharge method, first employed in the discovery of KrF₂, involves subjecting gaseous mixtures of krypton and fluorine (F₂) to high-energy electric arcs. Optimal conditions require a 1:1 to 2:1 molar ratio of F₂ to Kr at pressures of 40–60 torr (5.3–8.0 kPa) and low temperatures (−196°C) to stabilize the product . The reaction proceeds via plasma-induced dissociation of F₂ into atomic fluorine, which reacts with krypton atoms:
Kr+2F⋅→KrF2
Yield and Limitations
Early experiments achieved production rates of approximately 0.25 g/h, though yields remain inconsistent due to competing side reactions and energy losses . The method’s unreliability stems from:
-
Energy dissipation : Only a fraction of the applied electrical energy contributes to F₂ dissociation.
-
Thermal instability : KrF₂ decomposes rapidly above −30°C, necessitating cryogenic trapping .
Despite these challenges, electrical discharge remains historically significant as the first route to KrF₂ and its misidentified tetrafluoride analog .
Photochemical Synthesis
Ultraviolet Light Activation
Photochemical synthesis, pioneered by Lucia V. Streng and refined by J. Slivnik, utilizes UV light (303–313 nm) to excite F₂ molecules into dissociative states. The process is conducted at 77 K, where krypton exists as a solid and fluorine as a liquid, enhancing collision efficiency . Reaction vessels made of Pyrex (UV cutoff: 280 nm) outperform quartz (170 nm) due to selective filtering of high-energy photons, which otherwise degrade KrF₂ .
Production Efficiency
Under ideal conditions, photochemical methods yield up to 1.22 g/h, with material purity exceeding 95% . Key variables include:
-
Wavelength specificity : Optimal excitation occurs near 310 nm.
-
Phase control : Liquid F₂ ensures continuous fluorine supply to solid Kr .
A comparative study demonstrated hourly yields of 507 mg (Pyrex), 204 mg (Vycor), and 158 mg (quartz), highlighting the inverse relationship between UV energy and output .
Proton Bombardment
High-Energy Particle Acceleration
Proton bombardment involves irradiating Kr/F₂ mixtures with 10 MeV protons, typically generated by cyclotrons. The method achieves rapid KrF₂ formation at 133 K, with yields reaching 1 g/h . Proton interactions ionize F₂, generating reactive intermediates:
2 + \text{H}^+ \rightarrow \text{F}2^+ + \text{H}^\cdot \rightarrow 2\text{F}^\cdot + \text{H}^+F2+H+→F2++H⋅→2F⋅+H+
Practical Constraints
While efficient, this technique faces scalability issues due to:
-
Cyclotron dependency : Limited access to high-energy proton sources.
-
Radiation hazards : Handling radioactive byproducts requires specialized infrastructure .
Hot-Wire Technique
Thermal Dissociation of Fluorine
The hot-wire method employs resistive heating of a tungsten filament (680°C) to dissociate F₂ into atomic fluorine, which reacts with solid krypton . A temperature gradient of 900°C/cm between the wire and Kr substrate maximizes radical flux, achieving yields up to 6 g/h .
Electron Beam Irradiation
Cryogenic Radiolysis
Electron beam irradiation (1.5 MeV) of Kr/F₂ mixtures at −150°C produces crystalline KrF₂ with minimal decomposition . The process leverages secondary electrons to ionize F₂, facilitating radical-mediated synthesis:
F2+e−→2F⋅+e−
Stability and Output
Yields are comparable to proton bombardment (∼1 g/h), but the requirement for cryogenic containment and specialized accelerators restricts widespread use .
Comparative Analysis of Preparation Methods
Properties
CAS No. |
13773-81-4 |
---|---|
Molecular Formula |
F2K |
Molecular Weight |
121.79 g/mol |
IUPAC Name |
difluorokrypton |
InChI |
InChI=1S/F2Kr/c1-3-2 |
InChI Key |
QGOSZQZQVQAYFS-UHFFFAOYSA-N |
SMILES |
F[Kr]F |
Canonical SMILES |
F[Kr]F |
Key on ui other cas no. |
13773-81-4 |
Origin of Product |
United States |
Disclaimer and Information on In-Vitro Research Products
Please be aware that all articles and product information presented on BenchChem are intended solely for informational purposes. The products available for purchase on BenchChem are specifically designed for in-vitro studies, which are conducted outside of living organisms. In-vitro studies, derived from the Latin term "in glass," involve experiments performed in controlled laboratory settings using cells or tissues. It is important to note that these products are not categorized as medicines or drugs, and they have not received approval from the FDA for the prevention, treatment, or cure of any medical condition, ailment, or disease. We must emphasize that any form of bodily introduction of these products into humans or animals is strictly prohibited by law. It is essential to adhere to these guidelines to ensure compliance with legal and ethical standards in research and experimentation.