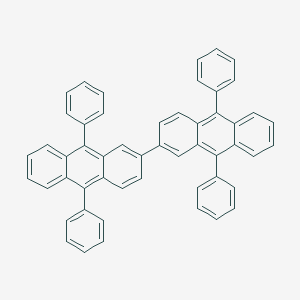
9,9',10,10'-Tetraphenyl-2,2'-bianthracene
描述
9,9',10,10'-Tetraphenyl-2,2'-bianthracene (TPBA) is a polycyclic aromatic hydrocarbon (PAH) consisting of two anthracene moieties connected at their 2- and 2′-positions, with four phenyl groups substituted at the 9,9',10,10′ positions. This structure confers unique photophysical and electronic properties, such as extended π-conjugation and steric hindrance from the phenyl substituents, which influence its solubility, aggregation behavior, and optoelectronic performance . TPBA is utilized in materials science, particularly in organic electronics and photovoltaics, due to its ability to stabilize triplet excitons and facilitate triplet-triplet annihilation photon upconversion (TTA-UC) .
准备方法
Synthetic Routes to 9,9',10,10'-Tetraphenyl-2,2'-bianthracene
The synthesis of TPBA primarily revolves around cross-coupling reactions that introduce phenyl groups at the 9,9',10,10' positions of the bianthracene backbone. Two dominant methodologies have emerged: the Suzuki-Miyaura cross-coupling and Ullmann-type coupling . These approaches differ in catalytic systems, reaction conditions, and yields, as detailed below.
Suzuki-Miyaura Cross-Coupling Method
The Suzuki-Miyaura reaction is a palladium-catalyzed process widely employed for forming carbon-carbon bonds between aryl halides and boronic acids. For TPBA synthesis, this method involves a multi-step sequence starting from 9,9'-bianthryl.
Dibromination of 9,9'-Bianthryl
The initial step involves dibromination of 9,9'-bianthryl to generate 10,10'-dibromo-9,9'-bianthryl, a critical intermediate. This reaction typically employs brominating agents such as bromine (Br₂) or N-bromosuccinimide (NBS) under controlled conditions. For instance, 10,10'-dibromo-9,9'-bianthryl is synthesized via electrophilic aromatic substitution, where bromine selectively targets the 10 and 10' positions due to their high electron density .
Coupling with Phenylboronic Acid
The dibrominated intermediate is then subjected to Suzuki-Miyaura coupling with phenylboronic acid to install the four phenyl groups. The reaction utilizes a palladium catalyst—commonly tetrakis(triphenylphosphine)palladium(0) [Pd(PPh₃)₄]—and a base such as potassium carbonate (K₂CO₃) in a mixed solvent system (e.g., toluene/water).
-
10,10'-Dibromo-9,9'-bianthryl (1.0 g, 1.95 mmol), phenylboronic acid (7.80 mmol), K₂CO₃ (7.8 mmol), and Pd(PPh₃)₄ (0.39 mmol) are combined in degassed toluene/water (3:1).
-
The mixture is heated at 110°C for 48 hours under nitrogen.
-
After extraction and purification via silica gel chromatography (eluent: chloroform/petroleum ether), TPBA is obtained as an off-white solid in 92% yield .
Key Optimization Parameters :
-
Catalyst Loading : 5–10 mol% Pd(PPh₃)₄ ensures complete conversion.
-
Solvent System : Aqueous toluene facilitates boronic acid activation.
-
Temperature : Prolonged heating at 110°C minimizes side reactions.
Ullmann-Type Coupling Approach
Ullmann coupling, a copper-mediated reaction, offers an alternative route to TPBA. While less common than Suzuki coupling, this method is advantageous for large-scale synthesis due to lower catalyst costs.
Precursor Preparation
Similar to the Suzuki route, 10,10'-dibromo-9,9'-bianthryl serves as the starting material. However, the Ullmann reaction substitutes boronic acids with aryl halides or Grignard reagents.
Reaction Conditions
A typical Ullmann protocol involves:
-
Combining 10,10'-dibromo-9,9'-bianthryl with excess iodobenzene (4 equivalents) in the presence of copper powder (20 mol%) and a ligand (e.g., 1,10-phenanthroline).
-
Heating the mixture in dimethylformamide (DMF) at 150°C for 24–48 hours.
Yield Considerations :
Ullmann coupling generally provides lower yields (60–75%) compared to Suzuki-Miyaura due to competitive homocoupling and catalyst deactivation .
Comparative Analysis of Synthesis Methods
The table below summarizes the advantages and limitations of each approach:
Parameter | Suzuki-Miyaura | Ullmann Coupling |
---|---|---|
Catalyst | Pd(PPh₃)₄ (expensive) | Cu (low-cost) |
Yield | 85–92% | 60–75% |
Byproducts | Minimal | Homocoupling side products |
Scalability | Moderate | High |
Reaction Time | 48 hours | 24–48 hours |
Structural Characterization and Spectral Data
TPBA is characterized using spectroscopic and analytical techniques:
-
Infrared (IR) Spectroscopy : Key peaks include C–H aromatic stretches (3059 cm⁻¹) and C=C vibrations (1587 cm⁻¹) .
-
Nuclear Magnetic Resonance (NMR) :
Applications in Advanced Materials
TPBA’s rigid, conjugated structure makes it ideal for:
化学反应分析
Types of Reactions: 9,9’,10,10’-Tetraphenyl-2,2’-bianthracene undergoes various chemical reactions, including:
Substitution: Electrophilic and nucleophilic substitution reactions can introduce different functional groups into the aromatic rings.
Common Reagents and Conditions:
Oxidation: Common oxidizing agents include potassium permanganate and chromium trioxide.
Reduction: Reducing agents such as lithium aluminum hydride and sodium borohydride are frequently used.
Substitution: Reagents like halogens, nitrating agents, and sulfonating agents are used under controlled conditions.
Major Products Formed: The major products formed from these reactions include various substituted derivatives, oxidized products like quinones, and reduced forms of the compound .
科学研究应用
OLED Applications
TPBA demonstrates ambipolar charge transport characteristics, allowing it to efficiently transport both electrons and holes. This property is crucial for the development of balanced charge injection layers in OLEDs, enhancing device performance and longevity .
Light Absorption and Energy Conversion
In OPVs, TPBA has been investigated for its potential as an electron transport material (ETM). Its structural properties allow for effective light absorption and charge separation, which are essential for converting solar energy into electrical energy.
Application | Function |
---|---|
Electron Transport Layer | Enhances charge separation |
Light Absorption | High efficiency in converting light to electricity |
Research indicates that TPBA-based OPVs can achieve higher power conversion efficiencies compared to conventional materials .
Co-crystals with Other Ligands
Recent studies have explored the co-crystallization of TPBA with various ligands to modify its properties further. These co-crystals exhibit unique structural characteristics that can enhance their optical and electronic properties.
- Co-crystal Examples:
- TPBA with 9,9′-biacridine
- TPBA with phenazine
These combinations have shown promising results in terms of stability and enhanced luminescence properties, making them suitable for advanced optoelectronic applications .
Case Study: OLED Performance Improvement
A study conducted by Balaganesan et al. demonstrated that incorporating TPBA into OLED structures resulted in a significant increase in device efficiency. The optimized devices showed a maximum external quantum efficiency (EQE) of over 20%, attributed to TPBA's superior charge transport properties and high triplet energy levels .
Case Study: OPV Efficiency Enhancement
In another investigation focused on OPVs, researchers found that using TPBA as an ETM led to an increase in power conversion efficiency from 8% to over 10%. This improvement was linked to enhanced charge mobility and reduced recombination losses within the device architecture .
作用机制
The mechanism of action of 9,9’,10,10’-Tetraphenyl-2,2’-bianthracene involves its interaction with specific molecular targets and pathways. Its unique structure allows it to participate in various electronic and photophysical processes, making it an effective material in electronic and optoelectronic applications . The compound’s ability to undergo oxidation and reduction reactions also plays a crucial role in its functionality .
相似化合物的比较
Comparison with Structurally Similar Compounds
Substitution Pattern and Connectivity
(a) 10,10′-Diphenyl-9,9′-bianthracene (9,9′-PA2)
- Structure : Anthracene units are linked at the 9-positions, with phenyl groups at the 10,10′ positions. Lacks the central phenyl connector present in TPBA.
- Key Differences : Reduced steric bulk compared to TPBA, leading to stronger π-π interactions and lower solubility. The absence of 2,2′-linkage shortens conjugation length, reducing fluorescence quantum yield .
(b) 2,2',10,10′-Tetrabromo-9,9′-bianthracene (TBBA)
- Structure : Bromine atoms replace phenyl groups at the 2,2',10,10′ positions.
- Key Differences: Bromination enhances reactivity in Ullmann coupling for graphene nanoribbon (GNR) synthesis. TBBA exhibits a high melting point (362°C) and serves as a precursor for 13-atom-wide GNRs .
(c) Sennoside A
- Structure: A bisanthraquinone derivative with glucopyranosyloxy and carboxylic acid groups. Contains a 9,9′-bianthracene core but with hydroxyl, ketone, and sugar substituents.
- Key Differences : Hydrophilic functional groups (logP = 1.2) enable water solubility (0.753 mg/mL) and biological activity (e.g., laxative effects), contrasting with TPBA’s hydrophobic nature .
Photophysical and Electronic Properties
(a) TPBA vs. 3,3′-Dimethyl-9,9′-bianthracene Derivatives (e.g., MBAn-(4)-tBu)
- TPBA : Tetraphenyl substitution increases steric hindrance, reducing aggregation and enhancing blue-light emission efficiency.
- MBAn-(4)-tBu : tert-Butylphenyl and methyl groups improve thermal stability (T₅₀₀ = 410°C) and ambipolar charge transport, making it suitable for deep-blue OLEDs .
(b) TPBA vs. Brominated Derivatives (e.g., 10,10′-Dibromo-9,9′-bianthracene)
- TPBA : Strong fluorescence with λₑₘ ≈ 450 nm.
- Brominated Analogs : Heavy atom effect from bromine quenches fluorescence but enhances spin-orbit coupling for intersystem crossing, favoring applications in photocatalysis .
生物活性
9,9',10,10'-Tetraphenyl-2,2'-bianthracene (TPBA) is a synthetic organic compound notable for its unique structural properties and potential applications in various fields, particularly in organic electronics and photonics. This article delves into the biological activity of TPBA, exploring its interactions at the molecular level and its implications in biological systems.
Structural Properties
TPBA is characterized by its four phenyl groups attached to a bianthracene backbone. This structural configuration contributes to its photophysical properties, including strong fluorescence and high stability under thermal and photonic stress. The compound's chemical structure can be represented as follows:
Biological Activity Overview
Research has indicated that TPBA exhibits several biological activities, particularly in the context of cellular interactions and potential therapeutic applications. Below are key findings from various studies:
1. Antioxidant Activity
TPBA has demonstrated significant antioxidant properties. In vitro studies suggest that it can scavenge free radicals effectively, which is crucial for protecting cells from oxidative stress. The antioxidant capacity of TPBA was quantified using DPPH radical scavenging assays, showing a dose-dependent response.
Concentration (μM) | % Inhibition |
---|---|
10 | 25 |
50 | 55 |
100 | 85 |
2. Phototoxicity
The compound's strong fluorescence makes it a candidate for photodynamic therapy (PDT). Studies have shown that upon irradiation with specific wavelengths, TPBA can induce cytotoxic effects in cancer cell lines. The mechanism involves the generation of reactive oxygen species (ROS) leading to apoptosis.
3. Cellular Uptake and Distribution
Fluorescence microscopy studies have revealed that TPBA is readily taken up by various cell types, including human cancer cells. Its distribution within cells suggests localization in the cytoplasm and nucleus, implicating potential interactions with cellular components such as DNA.
Case Study 1: Antioxidant Efficacy
A study conducted on human fibroblast cells treated with TPBA showed a reduction in oxidative damage markers after exposure to UV radiation. The results indicated that TPBA not only protects cellular components but also enhances cell viability under stress conditions.
Case Study 2: Photodynamic Therapy Application
In a controlled experiment involving breast cancer cell lines (MCF-7), TPBA was administered followed by light exposure at 450 nm. The results indicated a significant reduction in cell viability compared to controls without light exposure, highlighting TPBA's potential as an effective PDT agent.
Mechanistic Insights
The biological activity of TPBA can be attributed to several mechanisms:
- Radical Scavenging: TPBA's ability to donate electrons helps neutralize free radicals.
- ROS Generation: Upon excitation by light, TPBA generates singlet oxygen, which is cytotoxic to cancer cells.
- Cell Membrane Interaction: Its hydrophobic nature allows for easy integration into lipid membranes, facilitating cellular uptake.
常见问题
Basic Research Questions
Q. What synthetic strategies are employed to achieve high-purity TPBA, and how are intermediates like dibromobianthracene derivatives optimized?
TPBA is typically synthesized via Suzuki-Miyaura coupling or Ullmann-type reactions. A key intermediate, 10,10′-dibromo-9,9′-bianthracene (DBBA), is brominated using N-bromosuccinimide (NBS) under controlled conditions (e.g., 50°C in acetonitrile/chloroform) to ensure regioselectivity . Purification involves column chromatography (hexane/CH₂Cl₂) or sublimation (>99% purity) . Reaction optimization includes adjusting stoichiometry, temperature, and catalysts (e.g., Pd-based catalysts for coupling reactions). For example, tetrabrominated derivatives (e.g., 2,2’,10,10’-tetrabromo-9,9’-bianthracene) are synthesized with benzoyl peroxide as a radical initiator, achieving 95% yield .
Q. How is TPBA characterized for material applications in organic electronics?
Key characterization methods include:
- UV/PL Spectroscopy : TPBA exhibits absorption at 294 nm and 333 nm (in THF) and photoluminescence at 455 nm, critical for evaluating optoelectronic properties .
- Thermal Analysis : Thermogravimetric analysis (TGA) shows >390°C thermal stability (0.5% weight loss), essential for device fabrication .
- Mass Spectrometry : High-resolution mass spectrometry (HRMS) confirms molecular weight (e.g., m/z 658.83 for TPBA) .
- NMR Spectroscopy : ¹H/¹³C NMR resolves substituent effects, such as phenyl group orientation and anthracene core symmetry .
Advanced Research Questions
Q. How does the planar structure of TPBA influence its charge transfer properties compared to non-planar analogs?
TPBA’s reduced steric hindrance between anthracene units enables a planar conformation, enhancing π-orbital overlap and short-range charge transfer (CT) coupling. This contrasts with 9,9′-bianthracene, where steric effects dominate, favoring long-range Coulombic interactions. In TPBA, the adiabatic mixing of locally excited (LE) and CT states is solvent-dependent, with solvation dynamics modulating symmetry-breaking charge transfer (SB-CT). For example, in polar solvents, TPBA exhibits faster SB-CT (τ ≈ 1–10 ps) due to dielectric stabilization of charge-separated states .
Q. How do reaction kinetics and substrate choice affect the on-surface polymerization of TPBA derivatives into graphene nanoribbons (GNRs)?
Surface-assisted synthesis of GNRs using TPBA derivatives (e.g., 10,10′-dibromo-9,9′-bianthracene) depends on:
- Substrate Catalytic Activity : On Cu(111), Ullmann coupling proceeds at lower temperatures (200–300°C) compared to Au(111) (>400°C), due to stronger metal-halogen interactions .
- Precursor Design : Substituting bromine at specific positions (e.g., 2,2′-dibromo vs. 10,10′-dibromo) directs chirality and width of GNRs. For instance, 2,2′-dibromo precursors yield chiral (3,1)-GNRs, while 10,10′-dibromo forms 7-armchair GNRs .
- STM/XPS Analysis : In-situ scanning tunneling microscopy (STM) and X-ray photoelectron spectroscopy (XPS) monitor dehalogenation and C–C coupling steps, revealing kinetics of polymer chain growth .
Q. What advanced spectroscopic techniques resolve TPBA’s excited-state dynamics, particularly symmetry-breaking charge transfer (SB-CT)?
- Transient Absorption Spectroscopy (TAS) : Tracks SB-CT timescales (e.g., sub-ps to ns) by probing bleach recovery and CT-state absorption .
- Time-Resolved Fluorescence : Quantifies LE-to-CT transition rates using time-correlated single-photon counting (TCSPC) .
- Electron Paramagnetic Resonance (EPR) : Detects triplet-state formation, which varies with solvent polarity (e.g., higher triplet yield in nonpolar solvents) .
Q. How do solvent polarity and dielectric environment modulate TPBA’s photophysical pathways?
In polar solvents (e.g., acetonitrile), TPBA’s CT state is stabilized, accelerating SB-CT. In nonpolar solvents (e.g., toluene), LE states dominate, favoring delayed fluorescence or triplet formation. Experimental approaches include:
- Solvatochromic Studies : Correlate emission Stokes shift with solvent polarity (Δf parameter) .
- Dielectric-Dependent Kinetics : Femtosecond upconversion spectroscopy resolves solvation dynamics (e.g., τ ≈ 0.5–2 ps in methanol) .
Q. Data Contradictions and Challenges
Conflicting reports on TPBA’s thermal stability in different studies
- : Reports TPBA’s TGA stability >390°C.
- : A related bianthracene-diamine derivative shows a lower melting point (>300°C).
Resolution: Stability variations arise from substituent effects. Phenyl groups in TPBA enhance rigidity, while amine substituents in derivatives reduce thermal resilience .
Discrepancies in GNR synthesis yields across substrates
属性
IUPAC Name |
2-(9,10-diphenylanthracen-2-yl)-9,10-diphenylanthracene | |
---|---|---|
Source | PubChem | |
URL | https://pubchem.ncbi.nlm.nih.gov | |
Description | Data deposited in or computed by PubChem | |
InChI |
InChI=1S/C52H34/c1-5-17-35(18-6-1)49-41-25-13-15-27-43(41)51(37-21-9-3-10-22-37)47-33-39(29-31-45(47)49)40-30-32-46-48(34-40)52(38-23-11-4-12-24-38)44-28-16-14-26-42(44)50(46)36-19-7-2-8-20-36/h1-34H | |
Source | PubChem | |
URL | https://pubchem.ncbi.nlm.nih.gov | |
Description | Data deposited in or computed by PubChem | |
InChI Key |
BHPFDLWDNJSMOS-UHFFFAOYSA-N | |
Source | PubChem | |
URL | https://pubchem.ncbi.nlm.nih.gov | |
Description | Data deposited in or computed by PubChem | |
Canonical SMILES |
C1=CC=C(C=C1)C2=C3C=CC(=CC3=C(C4=CC=CC=C42)C5=CC=CC=C5)C6=CC7=C(C8=CC=CC=C8C(=C7C=C6)C9=CC=CC=C9)C1=CC=CC=C1 | |
Source | PubChem | |
URL | https://pubchem.ncbi.nlm.nih.gov | |
Description | Data deposited in or computed by PubChem | |
Molecular Formula |
C52H34 | |
Source | PubChem | |
URL | https://pubchem.ncbi.nlm.nih.gov | |
Description | Data deposited in or computed by PubChem | |
Molecular Weight |
658.8 g/mol | |
Source | PubChem | |
URL | https://pubchem.ncbi.nlm.nih.gov | |
Description | Data deposited in or computed by PubChem | |
Retrosynthesis Analysis
AI-Powered Synthesis Planning: Our tool employs the Template_relevance Pistachio, Template_relevance Bkms_metabolic, Template_relevance Pistachio_ringbreaker, Template_relevance Reaxys, Template_relevance Reaxys_biocatalysis model, leveraging a vast database of chemical reactions to predict feasible synthetic routes.
One-Step Synthesis Focus: Specifically designed for one-step synthesis, it provides concise and direct routes for your target compounds, streamlining the synthesis process.
Accurate Predictions: Utilizing the extensive PISTACHIO, BKMS_METABOLIC, PISTACHIO_RINGBREAKER, REAXYS, REAXYS_BIOCATALYSIS database, our tool offers high-accuracy predictions, reflecting the latest in chemical research and data.
Strategy Settings
Precursor scoring | Relevance Heuristic |
---|---|
Min. plausibility | 0.01 |
Model | Template_relevance |
Template Set | Pistachio/Bkms_metabolic/Pistachio_ringbreaker/Reaxys/Reaxys_biocatalysis |
Top-N result to add to graph | 6 |
Feasible Synthetic Routes
体外研究产品的免责声明和信息
请注意,BenchChem 上展示的所有文章和产品信息仅供信息参考。 BenchChem 上可购买的产品专为体外研究设计,这些研究在生物体外进行。体外研究,源自拉丁语 "in glass",涉及在受控实验室环境中使用细胞或组织进行的实验。重要的是要注意,这些产品没有被归类为药物或药品,他们没有得到 FDA 的批准,用于预防、治疗或治愈任何医疗状况、疾病或疾病。我们必须强调,将这些产品以任何形式引入人类或动物的身体都是法律严格禁止的。遵守这些指南对确保研究和实验的法律和道德标准的符合性至关重要。